Fast and slow feedforward inhibitory circuits for cortical odor processing
Figures
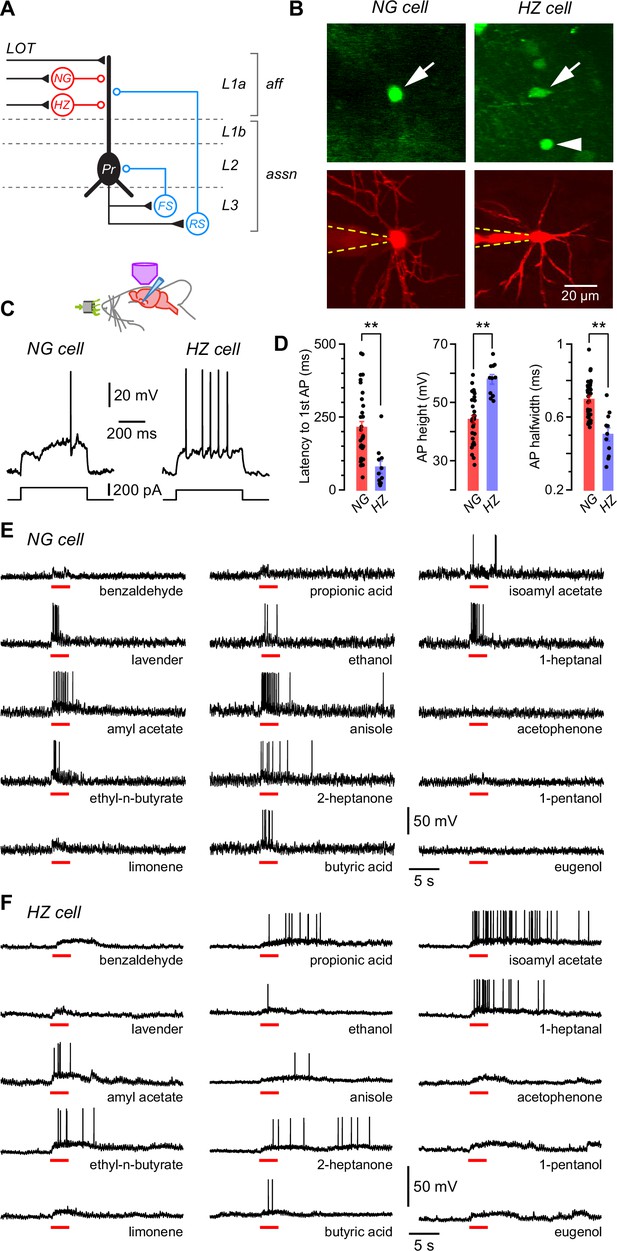
Layer 1a neurogliaform (NG) and horizontal (HZ) cells are reliably distinguished using two-photon-targeted patch clamping in vivo.
NG and HZ cells respond differently to odors. (A) Schematic showing two forms of synaptic inhibition received by principal cells (Pr) in the PCx: feedforward (red, mediated by NG and HZ cells in layer 1a [L1a]) and feedback (blue, mediated by fast-spiking [FS] and regular-spiking [RS] cells in L3). Feedback inhibition from other cell types (e.g. bitufted cells, deep NG cells) is not shown. LOT, lateral olfactory tract; aff, afferent layer; assn, associational layers. (B) (Top) Two-photon images from a GAD67-GFP (Δneo) mouse showing (arrowed, left) the very bright GFP fluorescence in an NG cell and the much weaker GFP in an HZ cell (arrowed, right; arrowhead indicates a nearby NG cell). (B) (Bottom) z-Projection of the same two cells imaged in the red channel; internal solution contained Alexa Fluor-594. Dashed yellow lines show approximate position of patch electrode. Scale bar (bottom right) applies to all panels. (C) Responses of NG cell (left) and HZ cell (right) in vivo to a depolarizing current step near rheobase. (D) Comparison of selected properties of action potentials recorded in NG and HZ cells in response to current steps in vivo. Points show data from individual neurons, bars show mean ± standard error of the mean (SEM). **, p < 0.01, n = 33 cells for NG, n = 11 cells for HZ, Welch’s two-sample unpaired t-test. (E) Response of an NG cell to a palette of 15 structurally diverse odors (name under each trace). Application period is shown by the red bar. Similar results were obtained from a total of 28 NG cells. (F) Recordings made from an HZ cell. Similar results were obtained from 10 HZ cells. Further details on analyzing these odor responses are in Figure 1—figure supplement 1.
-
Figure 1—source data 1
Excel spreadsheet listing intrinsic electrical data shown in Figure 1D.
This spreadsheet lists the mean and standard error of the mean (SEM) of the latency to first AP, AP height, and AP halfwidth for all neurogliaform (NG) and horizontal (HZ) cells in the dataset (shown as colored bars in Figure 1D) as well as the individual data for each cell (shown as points in Figure 1D). Additional parameters in the spreadsheet are not plotted.
- https://cdn.elifesciences.org/articles/73406/elife-73406-fig1-data1-v1.xlsx
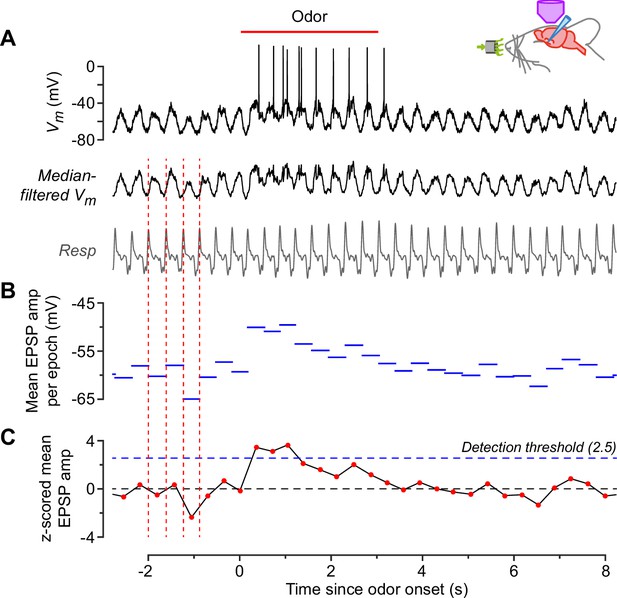
Measurement of odor responsiveness.
This example shows the response of a neurogliaform (NG) cell to ethyl-n-butyrate. (A) Raw membrane potential (Vm) (top) and Vm after median filtering to remove action potentials (middle). Bottom trace is the concurrently recorded respiration. Peaks in the respiration trace (corresponding to onset of exhalation) were detected (vertical dashed red lines) and the time between consecutive peaks was designated a ‘respiration epoch’. (B) Mean amplitude of the median-filtered Vm, averaged over each respiration epoch. (C) z-Scored version of the data in panel. (B) This was calculated by normalizing each point to the mean and standard deviation of the data points in (B) that occurred during the baseline period, that is, during the 8 s prior to odor application. If the z-score exceeded a detection threshold of 2.5 (horizontal dashed blue line) at any time during the odor application period, then an odor response was counted. This cell responded to 14 out of 15 odors. Each odor was applied only once to avoid habituation.
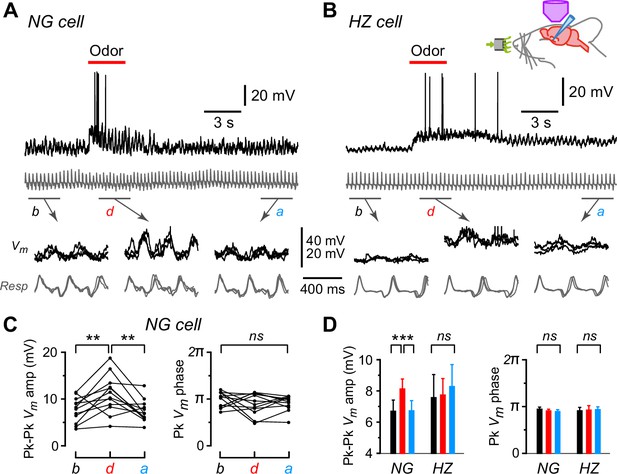
Oscillations in membrane potential (Vm) are synchronized to respiration in neurogliaform (NG) and horizontal (HZ) cells, and the oscillation amplitude is enhanced in NG cells during odors.
(A) (Top) Response of an NG cell to ethyl-n-butyrate. Gray trace below shows the respiration. (A) (Bottom) Indicated time windows are shown expanded, with the traces in three consecutive sub-windows shown superimposed: b, before odor application; d, during; a, after. (B) Same for the response of an HZ cell to ethyl-n-butyrate. (C) Summary of measurements from the NG cell in panel (A) when applying 12 different odors. Plots show peak-to-peak amplitude of mean Vm (left) and location of peak mean Vm expressed as phase of respiration cycle (right), each averaged over ~10 respiration cycles occurring in a 3-s-long window before, during, or after odor application. Lines connect measurements made in the same sweep for each of the 12 odors. (D) Summary of mean peak-to-peak Vm amplitudes (left) and phase (right) measured as in panel (C), averaged over n = 22 NG cells and n = 9 HZ cells. Black, red, and blue bars show mean ± standard error of the mean (SEM) before, during, and after odor application, respectively. ***, p < 0.001; **, p < 0.01; ns, not significant; one-way ANOVA with Tukey. Similar results were obtained using an alternative approach, cross-covariance analysis (Figure 2—figure supplement 1). In contrast, respiration-synchronized oscillations in Vm were not observed in an NG cell in the primary somatosensory cortex (Figure 2—figure supplement 2).
-
Figure 2—source data 1
Excel spreadsheet listing peak-to-peak amplitude, cross-covariance, and phase data shown in Figure 2D and Figure 2—figure supplement 1, panel D.
The sheet ‘PkPk Summary’ in this spreadsheet lists the mean peak-to-peak amplitude, peak phase, and respiration frequency before, during, and after odor application for each NG and HZ cell in the dataset, for which the population mean and standard error of the mean (SEM) are plotted in Figure 2D. Additional parameters listed in the spreadsheet are not plotted in Figure 2D. The sheet ‘CrCov Summary’ in this spreadsheet lists the cross-covariance amplitude and cross-covariance lag before, during, and after odor application for the same dataset. The population mean and SEM of these data are plotted in panel D of Figure 2—figure supplement 1.
- https://cdn.elifesciences.org/articles/73406/elife-73406-fig2-data1-v1.xlsx
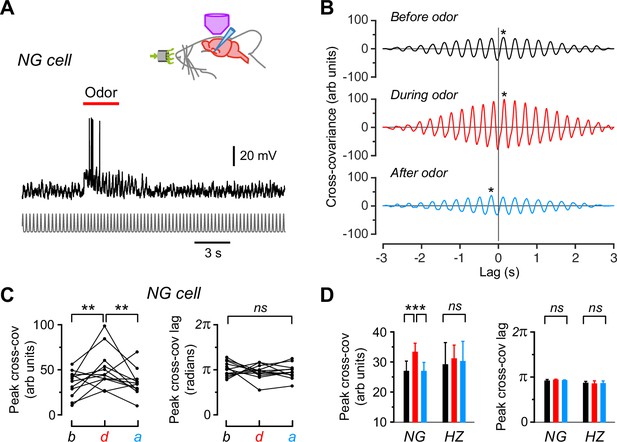
Cross-covariance analysis confirms the presence of respiration-locked oscillations in Vm in neurogliaform (NG) and horizontal (HZ) cells, with the amplitude of these oscillations increasing in NG cells during odors.
(A) Same NG cell as in Figure 2A, except that the respiration trace has been replaced by a normalized series of Gaussians representing the peaks in the respiration cycles (bottom). This was done to allow us to compare the cross-covariance between different cells by eliminating differences in the amplitude or shape of the respiration trace. (B) Cross-covariance between Vm and the Gaussian-normalized respiration for the cell in panel (A), calculated for 3-s-long windows starting 6 s before, 0.5 s after, and 16 s after odor onset, respectively. Asterisks indicate the peak cross-covariance found within ±0.3 s (approximately ±1 respiration cycle) of the zero lag time. (C) Changes in peak cross-covariance amplitude (left) and lag (right) before (b), during (d), and after (a) odor application for the cell in panel (A). Each triplet of connected points represents data obtained when applying a different odor to this cell. (D) Summary of average peak cross-covariance amplitude (left) and lag (right) for 22 NG cells and 9 HZ cells. Bars show mean ± standard error of the mean (SEM) (black, before odor; red, during; blue, after). ***, p < 0.001; **, p < 0.01; ns, not significantly different; one-way ANOVA with Tukey.
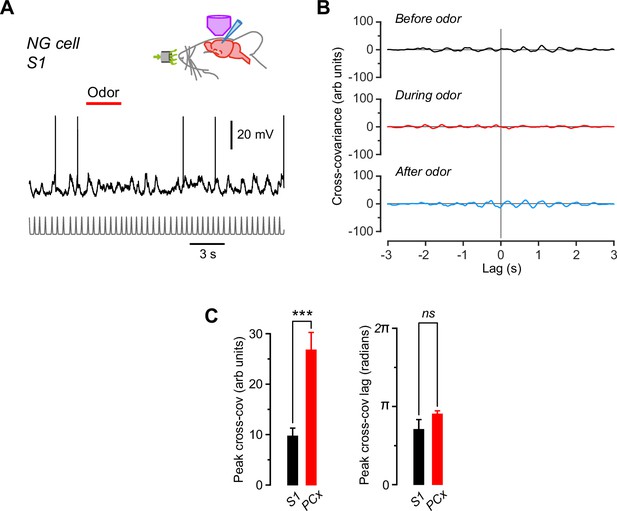
Subthreshold membrane potential (Vm) in neurogliaform (NG) cells in the primary somatosensory cortex (S1) does not oscillate in phase with respiration and does not respond to odors, in contrast to NG cells in the piriform cortex (PCx).
(A) Recording from an S1 NG cell while applying odor (here, lavender; red bar). Trace at bottom is a normalized series of Gaussians representing the peaks in the respiration cycles, as in Figure 2—figure supplement 1. (B) Cross-covariance between Vm and the Gaussian-normalized respiration for the cell in panel (A), calculated for 3-s-long windows starting 6 s before, 0.5 s after, and 16 s after odor onset, respectively. Traces are displayed on the same axes as for the piriform NG cell in Figure 2—figure supplement 1 (panel B) to facilitate comparison. Note the much weaker cross-covariance for this NG cell in S1. (C) Average peak cross-covariance amplitude (left) and lag (right) for the S1 NG cell in panels (A) and (B) (n = 11 trials) compared with the PCx NG cells (n = 22) in Figure 2—figure supplement 1D . ***, p < 0.001; ns, not significantly different; Welch’s two-sample unpaired t-test.
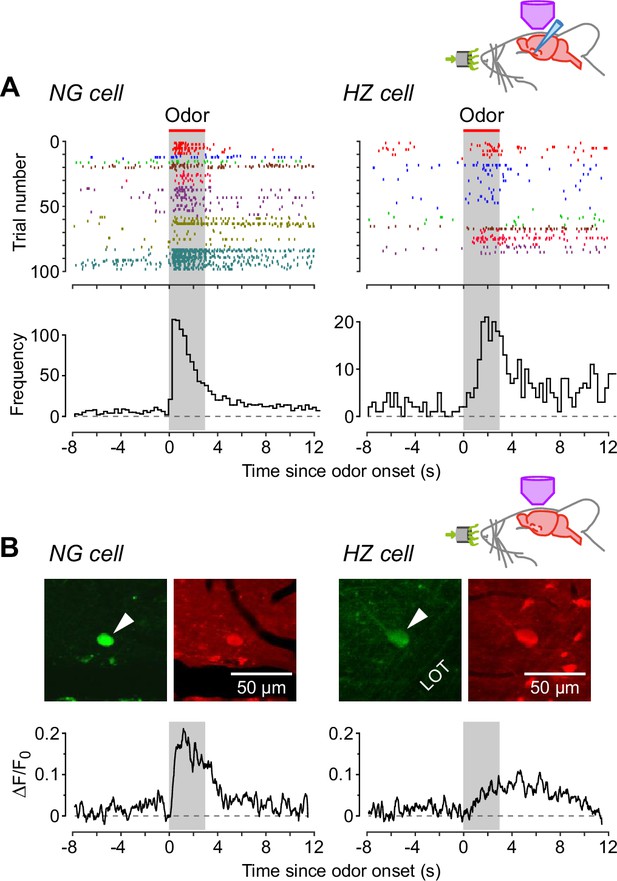
Neurogliaform (NG) cells tend to fire earlier than horizontal (HZ) cells in response to odors.
(A) (Top) Spike raster plots for NG cells (left) and HZ cells (right) in our dataset that fired at least one AP before, during, or after odor application (n = 98 trials in 8 NG cells, n = 86 trials in 6 HZ cells). Different colors indicate different cells. Gray bar indicates period of odor application. (A) (Bottom) Peristimulus time histograms (PSTHs) for the raster plots, showing the greater delay to odor-evoked firing in HZ cells. These responses were not limited by the rate of delivery of odorants by our olfactometer (Figure 3—figure supplement 1). (B) (Top) Two-photon images of GFP (green) and Cal-590 (red) fluorescence for a layer 1a NG cell (left, arrowhead) and an HZ cell (right, arrowhead). (B) (Bottom) Averaged ΔF/F0 plots for the somatic responses of n = 6 NG cells (left) and n = 5 HZ cells (right) in response to odor. Similar results were observed using alternative anesthesia that produces a more awake-like state (Figure 3—figure supplement 2).
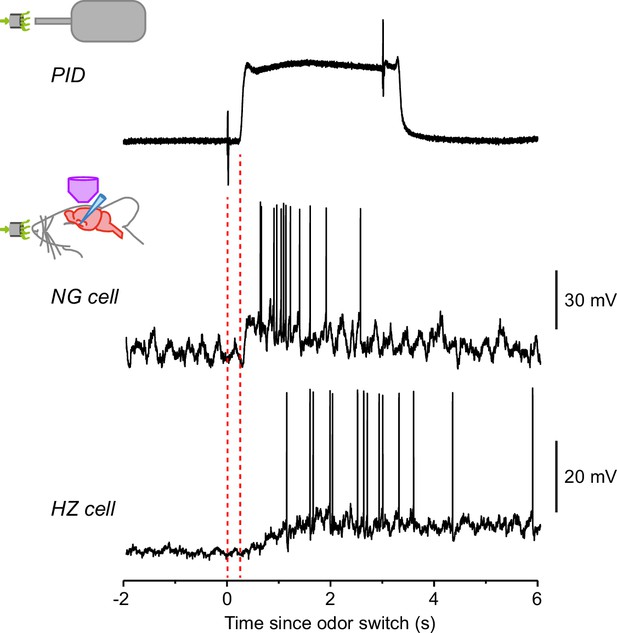
Response of horizontal (HZ) cells, but not neurogliaform (NG) cells, is much slower than the speed of odor arrival.
Top trace shows the odor application kinetics for our olfactometer, as measured using a photoionization detector (PID). The brief transients at times 0 and 3 s are due to the opening and closing, respectively, of the valve in the olfactometer. Note the delay to odor onset (242 ± 6 ms, n = 9 odors measured), and a similar delay at odor offset. (All data presented in this paper were corrected for this delay.) The 20–80% rise time of the odor arrival was 46 ± 2 ms (n = 9). The lower two traces show example recordings from an NG cell and an HZ cell, plotted on the same timescale as the PID trace. The NG cell response rises as rapidly as the odor onset, whereas the HZ response is delayed. These examples are taken from Figure 1 (1-heptanal).
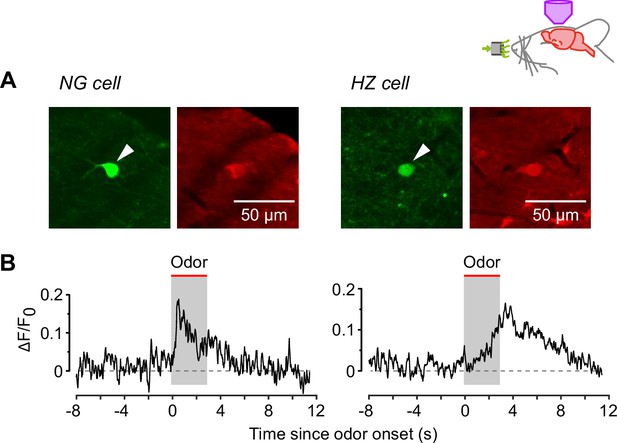
In vivo two-photon calcium imaging experiments confirm that neurogliaform (NG) and horizontal (HZ) cells respond similarly to odors in animals anesthetized with either urethane or fentanyl and medetomidine.
(A) In vivo two-photon images of GFP (green, arrowheads) and resting Cal-590 fluorescence (red) in an NG cell (left) and HZ cell (right). (B) Average add symbol F/F0 responses in n = 3 NG cells (left) and n = 5 HZ cells (right), recorded under fentanyl + medetomidine anesthesia. Odor was applied for the 3 s period indicated. The response kinetics are similar to those recorded under urethane anesthesia (Figure 3B).
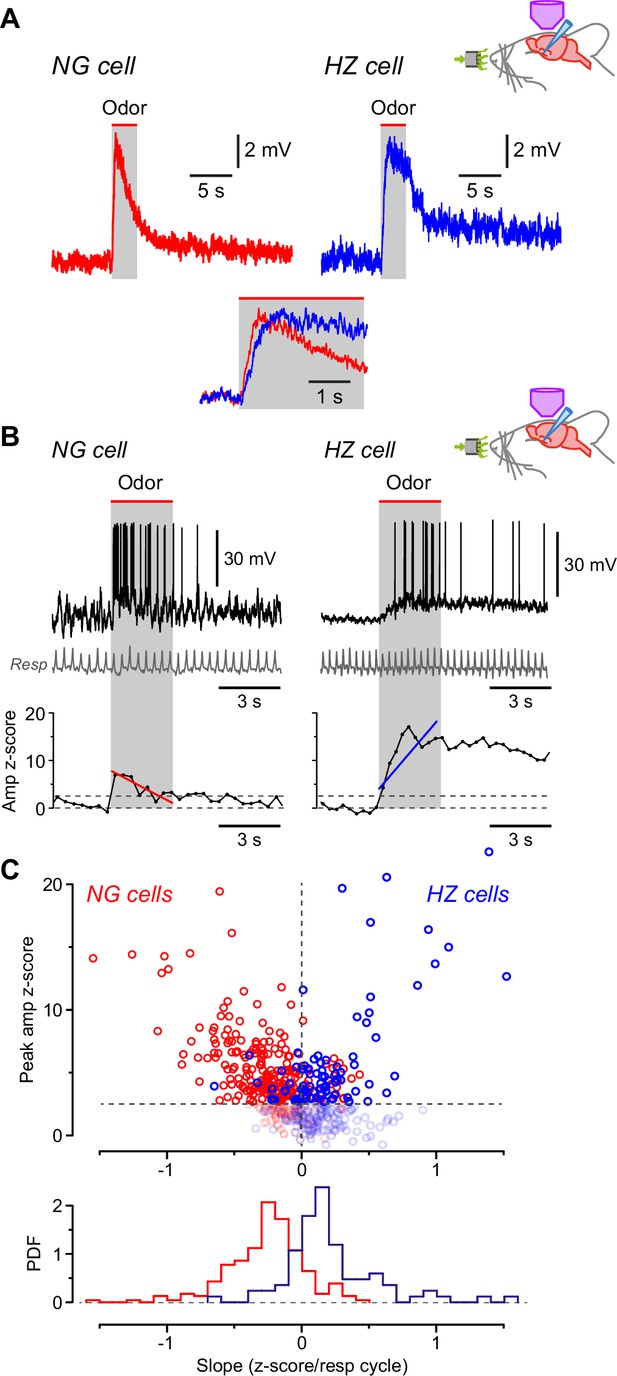
Odors cause Vm to depolarize more slowly in horizontal (HZ) cells than in neurogliaform (NG) cells.
(A) Averaged in vivo current clamp recordings of odor-evoked EPSPs measured in NG cells (left, n = 16 cells) and HZ cells (right, n = 6). Inset (bottom) shows the same traces normalized to their peaks, overlaid and expanded, showing that averaged EPSPs in HZ cells have a slower time to peak. To facilitate comparison, these EPSPs were filtered to remove respiration-linked oscillations (Figure 4—figure supplement 1). (B) (Top) Response of an NG cell to ethyl-n-butyrate (left) and an HZ cell to 1-heptanal (right). The corresponding respiration trace is shown below (gray). (B) (Bottom) z-Score-transformed mean Vm amplitude (averaged over each respiration cycle) calculated for the same two neurons as above. Spikes were removed before measuring Vm. Upper horizontal dashed line indicates the detection threshold (z-score = 2.5) for an odor-evoked response. Superimposed red (left) or blue (right) line represents a linear fit to the data points over the 3-s-long odor application period (gray band), giving slopes of –0.70 and 1.36 z-score units/respiration cycle, respectively. (C) (Top) Plot of peak Vm z-score during odor application versus slope (fitted as in panel B, bottom), where each data point represents the odor response of a single cell (red symbols, n = 310 responses from 28 NG cells; blue symbols, n = 188 responses from 9 HZ cells). Points below the threshold for an odor-evoked response (z-score = 2.5) have been grayed out. (C) (Bottom) Probability density function (PDF) of the points plotted in panel (C) (top), excluding the grayed-out values. NG cells (red plot) show a significant skew toward negative slopes, whereas HZ cells (blue) skew toward positive slopes (p < 0.001; Kolmogorov-Smirnov test).
-
Figure 4—source data 1
Excel spreadsheet listing z-score-transformed peak Vm during odor application and slope, shown in Figure 4C.
- https://cdn.elifesciences.org/articles/73406/elife-73406-fig4-data1-v1.xlsx
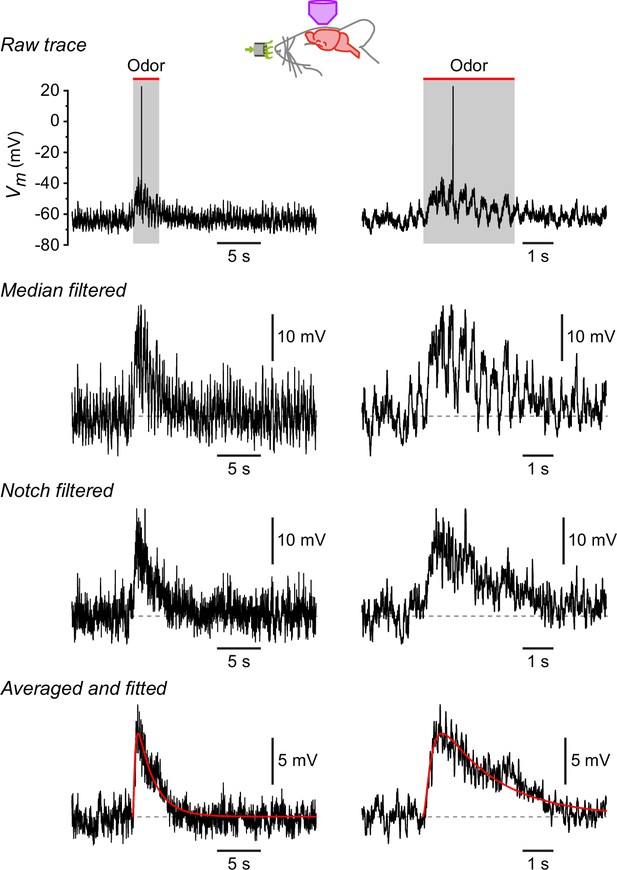
Procedure for measuring the properties of odor-evoked EPSPs.
Traces show, from top to bottom, the cumulative effect of each step in the analysis. Each row shows the same trace on two different timescales. Raw trace, response of a neurogliaform (NG) cell to lavender. Median filtered, same trace after median filtering to remove the action potential. Notch filtered, same trace after notch filtering at 2–4 Hz to remove the respiration-synchronized oscillations in Vm. Averaged and fitted, average of responses of this cell to eight different odorants, each response filtered as above, then fitted to a smooth function of the form m2h (superimposed red curve). The mean EPSP time to peak and halfwidth were measured from the fitted curve.
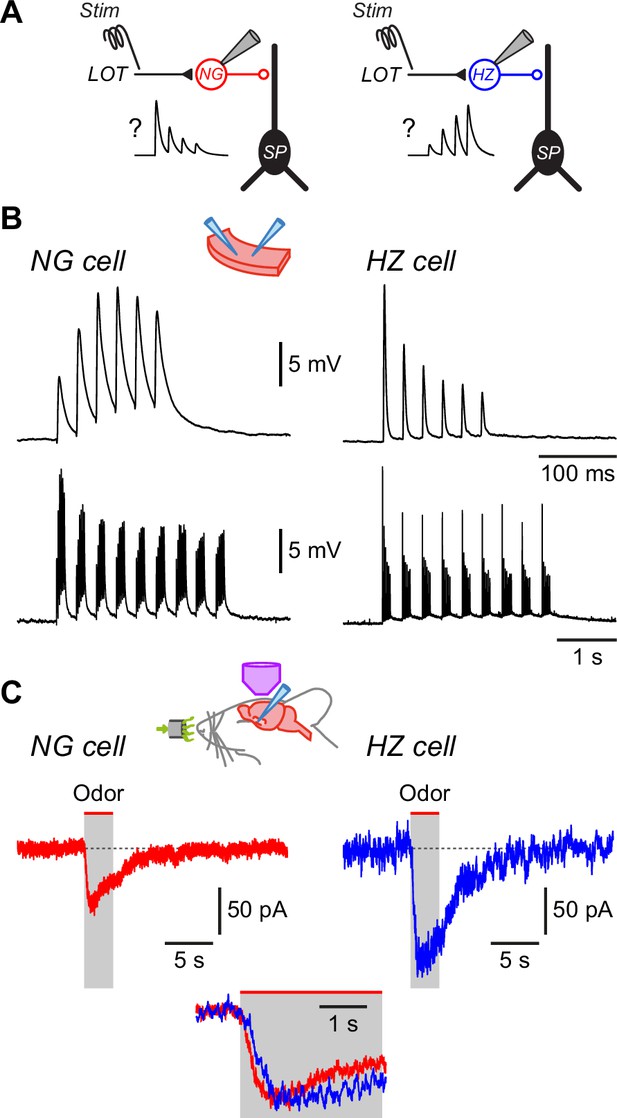
Differences in EPSC kinetics partly account for the different odor responsiveness of neurogliaform (NG) and horizontal (HZ) cells.
(A) Schematic showing the circuit dynamics that are proposed to explain the results in Figure 4, viz. lateral olfactory tract (LOT) input onto NG and HZ cells is hypothesized to be depressing or facilitating, respectively, as illustrated in the schematic EPSP traces labelled ‘?’. (B) Slice experiments showing EPSPs elicited in NG cells and HZ cells in response to trains of extracellular stimuli applied to the LOT with 100 µM picrotoxin in the bath to block GABAA receptors. (B) (Top) Six stimuli at 40 Hz elicit facilitating EPSPs in an NG cell (left) but depressing EPSPs in an HZ cell (right), which is opposite to the hypothesized responses (panel A).(B) (Bottom) A longer train (9 bursts of 40 Hz trains, repeated at 3 Hz), thought to more closely replicate odor-evoked stimuli, elicits a depressing envelope of EPSPs in both an NG cell (left) and an HZ cell (right). Thus, the hypothesis in panel (A) is supported for NG cells but not for HZ cells. Stimulus artifacts are blanked. Similar results were obtained in n = 6 experiments of this kind for each cell type. (C) Averaged in vivo voltage clamp recordings of odor-evoked EPSCs measured in NG cells (left, n = 17 cell-odor pairs) and HZ cells (right, n = 16 cell-odor pairs). Holding potential –70 mV. Inset (bottom) shows the same traces overlaid and expanded, showing that averaged EPSCs in HZ cells have a slower time to peak.
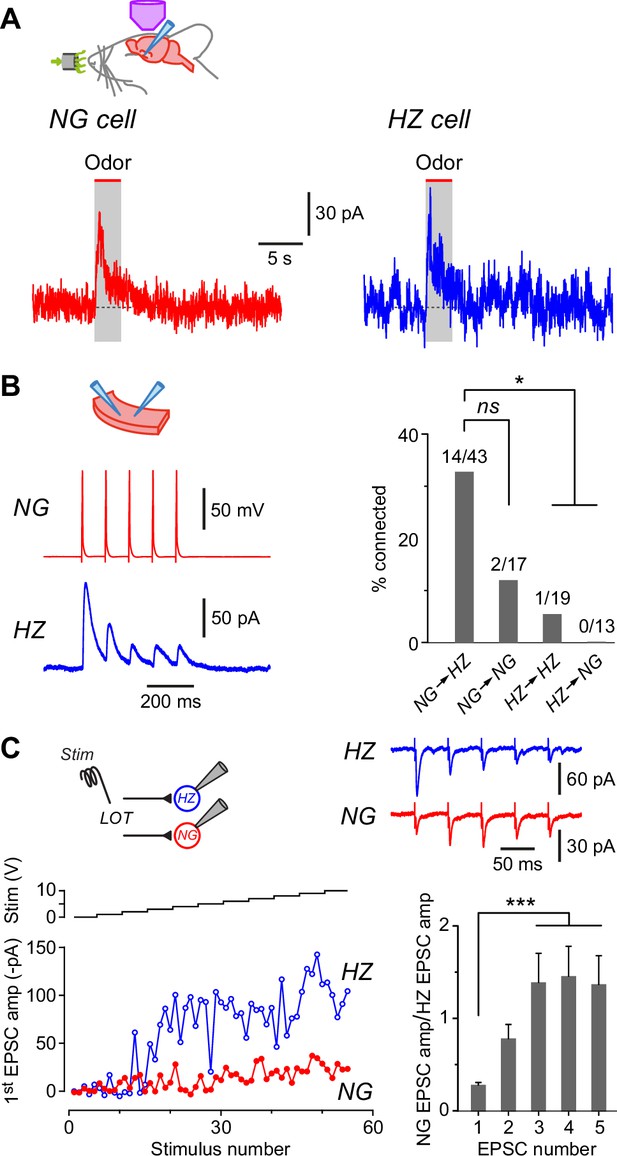
Neurogliaform (NG) and horizontal (HZ) cells receive odor-evoked feedforward inhibition, predominantly from other NG cells.
(A) Averaged in vivo voltage clamp recordings of odor-evoked IPSCs measured in NG cells (left, n = 14 cell-odor pairs) and HZ cells (right, n = 5 cell-odor pairs). Holding potential +10 mV. In separate experiments it was found that synaptic inhibition could also be observed in HZ cells under current clamp (Figure 6—figure supplement 1). (B) (Left) Dual recording from a synaptically connected NG cell and HZ cell pair in a slice. Stimulus was a 20 Hz train of 2-ms-long depolarizing current steps applied to the NG cell. Postsynaptic response is an average of 15 episodes (holding potential, 0 mV). (B) (Right) Summary bar plot showing percentage of NG/HZ cell pairs tested in vitro that were synaptically connected. Numbers above bars indicate ‘number of connections/total number of pairs tested’. *, p < 0.05; ns, not significant; chi-square 2 × 2 contingency test. (C) (Top left) Schematic showing the recording configuration for this panel, with a stimulating electrode in the lateral olfactory tract (LOT) and dual whole-cell recordings from an NG cell and an HZ cell in a slice. (C) (Bottom left) Example experiment showing peak EPSC amplitude for the first EPSC in a train of five recorded simultaneously in NG and HZ cells plotted versus stimulus number (red and blue traces, bottom) while increasing the stimulus strength in 1 V steps (‘Stim’, black staircase, top). (C) (Top right) Example data for the experiment shown in bottom left. Each trace is the average of responses to stimulus numbers 30–39. Stimulus artifacts are truncated. (C) (Bottom right) Summary bar plot showing the mean ± standard error of the mean (SEM) of NG:HZ EPSC amplitude ratios for all recorded pairs calculated for each EPSC in the train. ***, p < 0.001, n = 7 dual recordings in 7 slices from 4 mice.
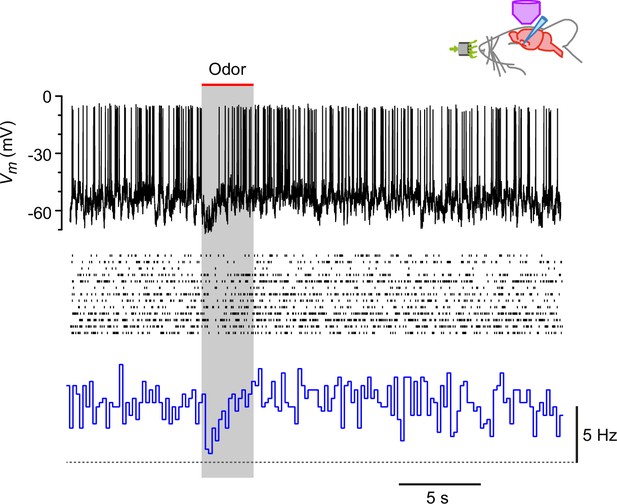
Steady depolarization of a horizontal (HZ) cell in vivo reveals odor-evoked hyperpolarization and suppression of firing.
Recordings from an HZ cell that has been depolarized by steady current injection then presented with 13 different odors. Top, example showing the transient inhibition of spiking during odor presentation (here, lavender). Middle, raster plots from all 13 odor presentations to this cell. Bottom, PSTH of the data in the middle panel, showing that the steady spiking (~5 Hz) is transiently inhibited by odor. Horizontal dashed line indicates 0 Hz baseline.
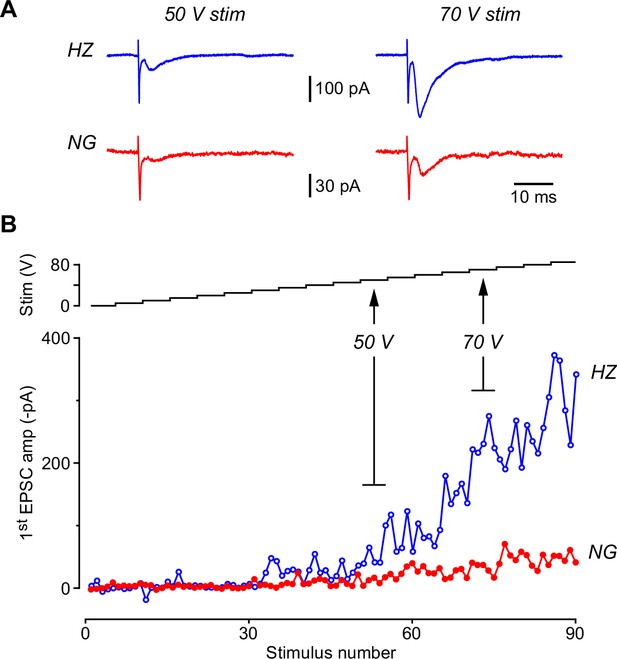
The lateral olfactory tract (LOT)-evoked EPSC recorded in a horizontal (HZ) cell is larger than that recorded simultaneously in a neurogliaform (NG) cell even when a ‘plateau’ is not apparent in the stimulus-response plot.
(A) Example EPSCs recorded simultaneously in an HZ cell (blue traces, top) and an NG cell (red traces, bottom) at two different stimulus strengths (50 V, left; 70 V, right). The first EPSC in a train of 5 is shown. Each trace is the average of five individual episodes measured at that stimulus strength, as indicated in panel (B). Stimulus artifacts have been truncated. (B) (Bottom) Stimulus-response plot showing the amplitude of the first EPSC plotted versus stimulus number as the stimulus strength is increased from 0 to 85 V in steps of 5 V, as indicated by the staircase plot in the top panel. Five paired EPSCs are evoked at each stimulus setting. Same experiment as in panel (A).
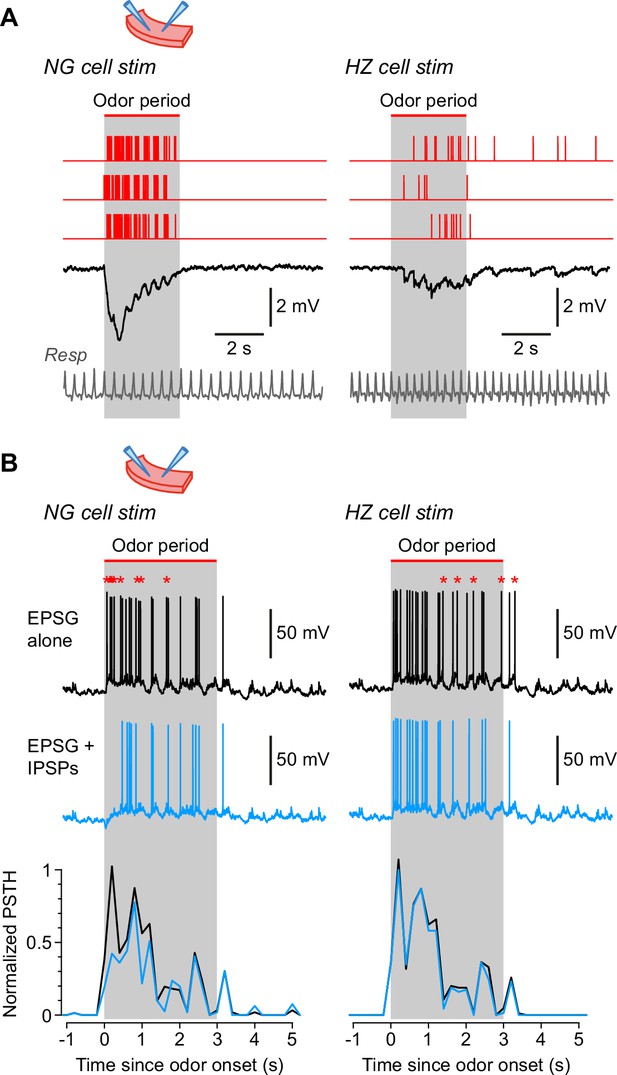
Neurogliaform (NG) and horizontal (HZ) cells produce different patterns of synaptic inhibition in postsynaptic superficial pyramidal (SP) cells.
(A) Average IPSPs recorded in vitro in an SP cell (black traces; stimulus artifacts blanked) in response to extracellular stimulation in layer 1a of the slice, using spiking patterns that had previously been recorded in vivo in either NG cells (left) or HZ cells (right). Examples of these spiking patterns are shown in the red traces (top). IPSPs were averaged from n = 6 SP cells, to each of which was applied up to 16 different stimulus patterns (NG and HZ). To preserve possible respiration-synchronized effects (Figure 2) when averaging across different stimulus patterns, each raw IPSP trace was ‘warped’ to match a reference respiration trace (gray, bottom). (B) (Top) Example action potentials recorded in SP cells while using a dynamic clamp to inject an odor-evoked excitatory postsynaptic conductance (EPSG) that had previously been recorded in vivo (data in Figure 7—source code 1). Responses with the EPSG alone (upper traces, black) were interleaved with responses to the EPSG plus extracellular synaptic stimulation (lower traces, blue; stimulus artifacts blanked) driven by different odor-evoked spiking patterns for NG cells (left) or HZ cells (right). As in panel (A), stimulus patterns were warped to a reference respiration trace (Figure 7—source code 1). Asterisks above the upper traces label action potentials that are absent in the lower traces. All slice recordings in panels (A) and (B) were made in the presence of 20 µM CNQX and 25 µM D-AP5. (B) (Bottom) Mean normalized PSTH plots from n = 7 SP cells showing that synaptic inhibition patterned on NG cell activity significantly suppresses early firing in SP cells (left), whereas inhibition patterned on HZ cell firing has a weak, delayed effect (right). Gray bars labelled ‘odor period’ in panels (A) and (B) indicate the period during which odor was applied in the in vivo recordings from NG or HZ cells. These recordings were used to construct the in vivo-like stimulus patterns (sample data in Figure 7—source code 1).
-
Figure 7—source code 1
Igor procedure file containing code used to acquire the data in Figure 7, as well as sample data and a ReadMe file explaining how to install and run the code.
- https://cdn.elifesciences.org/articles/73406/elife-73406-fig7-code1-v1.zip
Tables
Reagent type (species) or resource | Designation | Source or reference | Identifiers | Additional information |
---|---|---|---|---|
Genetic reagent (Mus musculus) | GAD67-GFP (Δneo) | Tamamaki et al., 2003, https://doi.org/10.1002/cne.10905 | GAD67-GFP mice have the EGFP gene targeted to the Gad1 locus. Breeders were obtained from Tamamaki et al., 2003. The colony was maintained on a C57BL6/J background | |
Commercial assay or kit | ABC kit | Vector Laboratories | Vectastain Elite ABC Kit,Cat # PK-6100 | |
Chemical compound, drug | Urethane | Merck/Sigma-Aldrich | Cat # U2500-100G | 0.7 g/kg s.c. |
Chemical compound, drug | Chlorprothixene | Merck/Sigma-Aldrich | chlorprothixene hydrochloride,Cat # C1671-1G | 5 mg/kg i.p. |
Chemical compound, drug | Atropine | Apex Laboratories, Australia | Atropine sulphate, 0.6 mg/ml | 0.2–0.3 mg/kg s.c. |
Chemical compound, drug | Alexa Fluor 594 | Life Technologies | Alexa Fluor 594 hydrazide, Na salt, Cat # A-10438 | 20 µM |
Chemical compound, drug | Acetophenone | Merck/Sigma- Aldrich | Cat # A10701-5ML | Flow dilution to 10% of saturated vapor pressure |
Chemical compound, drug | Anisole | Merck/Sigma-Aldrich | Cat # 96109 | Flow dilution to 10% of saturated vapor pressure |
Chemical compound, drug | Benzaldehyde | Merck/Sigma-Aldrich | Cat # 418099 | Flow dilution to 10% of saturated vapor pressure |
Chemical compound, drug | Butyric acid | Merck/Sigma-Aldrich | Cat # 19215–5ML | Flow dilution to 10% of saturated vapor pressure |
Chemical compound, drug | Ethanol | Merck/Sigma-Aldrich | Cat # 459836 | Flow dilution to 10% of saturated vapor pressure |
Chemical compound, drug | Ethyl-n-butyrate | Merck/Sigma-Aldrich | Cat # 75563 | Flow dilution to 10% of saturated vapor pressure |
Chemical compound, drug | Eugenol | Merck/Sigma-Aldrich | Cat # 35995 | Flow dilution to 10% of saturated vapor pressure |
Chemical compound, drug | 1-Heptanal | Merck/Sigma-Aldrich | Cat # 61696 | Flow dilution to 10% of saturated vapor pressure |
Chemical compound, drug | 2-Heptanone | Merck/Sigma-Aldrich | Cat # 02476 | Flow dilution to 10% of saturated vapor pressure |
Chemical compound, drug | Lavender oil | Merck/Sigma-Aldrich | Cat # 61718 | Flow dilution to 10% of saturated vapor pressure |
Chemical compound, drug | Limonene | Merck/Sigma-Aldrich | Cat # 62118 | Flow dilution to 10% of saturated vapor pressure |
Chemical compound, drug | 1-Pentanol | Merck/Sigma-Aldrich | Cat # 77597 | Flow dilution to 10% of saturated vapor pressure |
Chemical compound, drug | Propionic acid | Merck/Sigma-Aldrich | Cat # 94425 | Flow dilution to 10% of saturated vapor pressure |
Chemical compound, drug | Amyl acetate | MP Biomedicals | Cat # 300015 | Flow dilution to 10% of saturated vapor pressure |
Chemical compound, drug | Isoamyl acetate | MP Biomedicals | Cat # 155077 | Flow dilution to 10% of saturated vapor pressure |
Chemical compound, drug | DNQX | Tocris | 6,7-Dinitroquinoxaline-2,3-dione,Cat # 0189 | 10 µM |
Chemical compound, drug | D-AP5 | Tocris | D-aminophosphono valeric acid,Cat # 0106 | 50 µM |
Chemical compound, drug | Picrotoxin | Merck/Sigma-Aldrich | Cat # P1675 | 100 µM |
Chemical compound, drug | SR 95531 (gabazine) | Hello Bio | Cat # HB0901 | 20 µM |
Software, algorithm | Igor Pro | Wavemetrics; source code for dynamic clamp procedure is in Figure 7—source code 1 | https://www.wavemetrics.com/ | Source code folder (Figure 7—source code 1) also contains sample data |
Software, algorithm | Axograph X | Axograph Scientific | https://axograph.com/ | |
Software, algorithm | Matlab | Mathworks | https://www.mathworks.com/ | |
Software, algorithm | RStudio | RStudio | https://www.rstudio.com/ | |
Other | Cal-590 AM | AAT Bioquest | Cat # 20511 (10× 50 µg) | 1 mM (pressure-injected) |