BK channel properties correlate with neurobehavioral severity in three KCNMA1-linked channelopathy mouse models
Figures
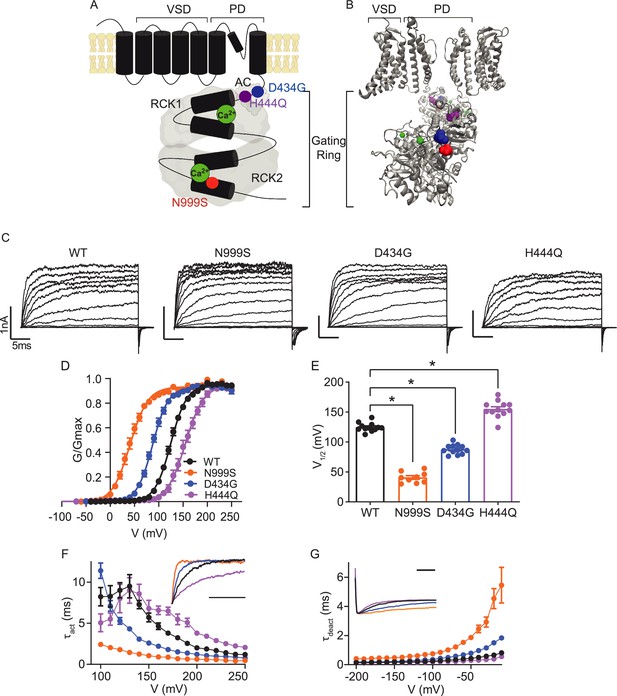
Location and consequence of KCNMA1 variants in the BK K+ channel.
(A) KCNMA1 forms the homotetrameric BK channel. Each α subunit is comprised of seven transmembrane domains (S0‒S6) and an intracellular gating ring with hydrophobic segments (S7–S10, black). Pore (+) opening and closing is regulated by voltage-sensitive residues in S2‒S4 (VSD), the AC domain (βA to αC), and two Regulators of Conductance of Potassium (RCK) domains in the gating ring (gray), each containing a Ca2+ binding site (green) (Yang et al., 2015; Giraldez and Rothberg, 2017). (B) BK channel structure showing two opposing subunits with Ca2+ bound in the gating ring (PDB 6V38). H444Q (purple) and D434G (blue) are located within the βB-αB and αA and βB of the AC domain, respectively, a region within RCK1 affecting Ca2+-dependent gating (Du et al., 2005; Tao and MacKinnon, 2019). N999S (red) is located at the helix bend in the middle of the S10 domain within RCK2 (Tao and MacKinnon, 2019). (C) Representative inside-out patch-clamp recordings from BKWT, BKN999S, BKD434G, BKH444Q channels expressed in HEK293 cells. Macroscopic BK currents were recorded in symmetrical K+ and 1 μM intracellular Ca2+ by holding patches at −100 mV, stepping from −100 to 250 mV for 30 ms, followed by a tail step −100 mV for 15 ms. Scale bars: 1 nA, 5 ms. (D) Normalized conductance-voltage (G-V) relationships fit with Boltzmann functions (solid lines). There was no change in the slope factor (z) for any of the variants (p=0.06, one-way ANOVA). BKWT (n=12), BKN999S (n=9), BKD434G (n=12), and BKH444Q (n=12). (E) Voltage of half-maximal activation (V1/2) obtained from Boltzmann fits for individual patches. *p<0.0001. One-way ANOVA with Dunnett’s post hoc. (F) Activation time constants (τact). BKN999S and BKD434G channels had decreased τact compared to BKWT, either across all voltage steps (mixed effects model for repeated measures with Bonferroni post hoc, p<0.01) or above 120 mV (p<0.05), respectively. At lower voltages, BKD434G channels were more steeply voltage dependent, but did not exceed the fast activation time constants of BKN999S channels. BKH444Q channels had increased τact compared to BKWT between 160 and 250 mV (p<0.05). Inset: Representative current traces from 170 mV step, scaled to the maximal current to illustrate activation timecourse (x-axis scale bar: 10 ms). τact for BKWT currents was 3.8±0.3 ms, while BKN999S and BKD434 currents activated faster (0.9±9.1 and 1.8±0.1 ms, respectively) and BKH444Q activated slower (6.5±0.5 ms). (G) Deactivation time constants (τdeact). BKN999S and BKD434G channels had increased τdeact compared to BKWT, across all voltage steps (mixed effects model for repeated measures with Bonferroni post hoc, p<0.01), with the exception of ‒160 (p>0.05), respectively. BKH444Q channels had decreased τdeact compared to BKWT between ‒190 mV and between ‒140 and ‒20 mV (p<0.05). Inset: Representative current traces from ‒20 mV step, scaled to the maximal current to illustrate deactivation timecourse (x-axis scale bar: 10 ms). τdeact for BKWT currents was 0.7±0.01 ms, while BKN999S and BKD434 currents deactivated slower (4.5±0.7 and 1.5±0.1 ms, respectively) and BKH444Q deactivated more quickly (0.4±0.01 ms). Data are presented as mean ± SEM. Additional data on the effects of stimulants on BKWT and BKN999S channels appears in Figure 1—figure supplement 1.
-
Figure 1—source data 1
Data file for Figure 1D–G.
- https://cdn.elifesciences.org/articles/77953/elife-77953-fig1-data1-v1.xls
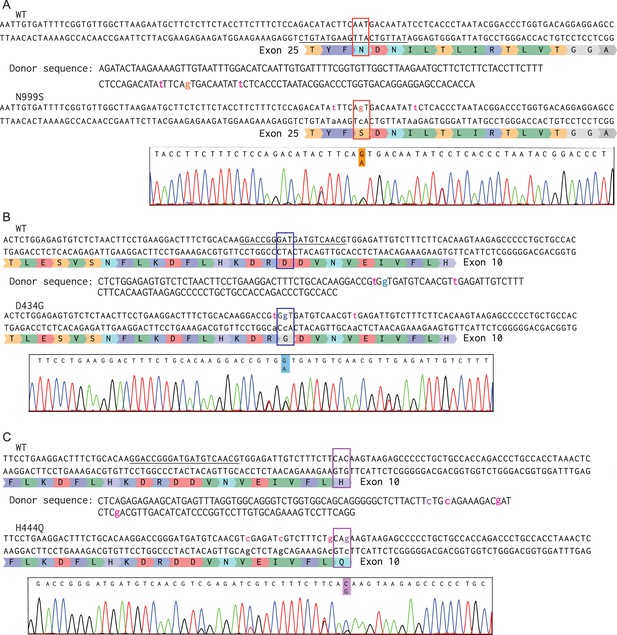
Sequences for CRISPR/Cas9 editing of the mouse Kcnma1 gene.
(A) Kcnma1N999S/WT mice were generated by introducing a non-synonymous mutation within the codon AAT→ AgT (red boxes) in exon 25. WT sequence is C57BL/6J. Underlined nucleotides are the gRNA sequence. Lowercase letters denote mutations. Chromatogram from an N1 heterozygous mouse. (B) Kcnma1D434G/WT mice were generated by mutation within the codon GAT→ GgT in exon 10. Chromatogram from a founder mouse. (C) Kcnma1H444Q/WT mice were generated by mutation within the codon CAC → CAg in exon 10 (same guide RNA as D434G). Chromatogram from a founder mouse.
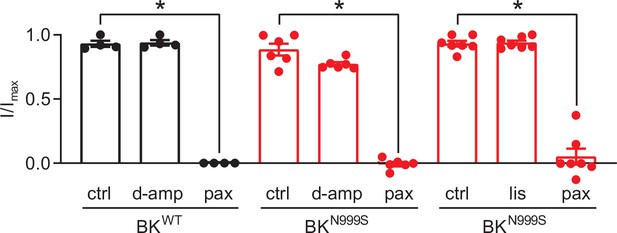
Effects of lisdexamfetamine (lis) and dextroamphetamine (d-amp) on BKWT and BKN999S channels expressed in HEK293 cells.
D-amp and lis, a prodrug of D-amphetamine and L-lysine, have been reported to treat paroxysmal non-kinesigenic dyskinesia (PNKD) episodes in six children harboring N999S variants, as well as one child harboring another gain-of-function (GOF) variant (Miller et al., 2021; Zhang et al., 2020). To test if either drug has a direct effect on BK currents in heterologous cells, as has been suggested for methamphetamine (Lin et al., 2016; Fu et al., 2021; Tatro et al., 2013; Wang et al., 2013), d-amp and lis were applied to patches from HEK293 cells expressing BKWT or BKN999S. Macroscopic BK currents were evaluated after perfusion of each drug and compared to pre-drug control current levels. Normalized data presented as the proportion of the maximal current (I/Imax) for each patch, before (control) and after drug application. Neither 155 ng/ml lis (BKN999S: n=7, p=0.98; one-way ANOVA) nor 155 ng/ml d-amp (BKWT: n=4, p=0.99; one-way ANOVA and BKN999S: n=6, p=0.15; one-way ANOVA) produced a decrease in BK current levels. However, the BK channel inhibitor paxilline (pax, 100 nM), applied as a control, fully abrogated BKWT or BKN999S currents at the end of each experiment (p<0.001; one-way ANOVA for all). Data are presented as mean ± SEM.
-
Figure 1—figure supplement 2—source data 1
Data file for Figure 1—figure supplement 2.
- https://cdn.elifesciences.org/articles/77953/elife-77953-fig1-figsupp2-data1-v1.xls
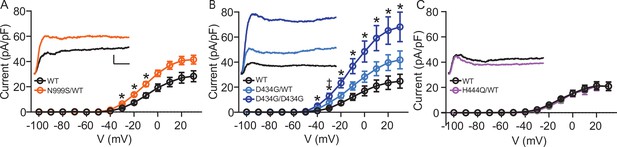
Increased BK current in Kcnma1N999S/WT and Kcnma1D434G/D434G granule neurons.
Whole-cell macroscopic BK currents were recorded in 1 μM tetrodotoxin (TTX) and 2 mM 4-aminopyridine (4-AP), isolated with 10 μM paxilline, and normalized to cell capacitance. Activating voltage steps were applied from Vh of ‒90 mV, stepping from ‒100 to +30 mV for 150 ms, and back to ‒90 mV for 130 ms. (A‒C) Peak BK current density versus voltage relationships. Data are presented as mean ± SEM. * and †, p<0.05, two-way repeated measures ANOVA with Bonferroni post hoc. Insets: Representative BK current traces at 30 mV. Scale bars: 500 pA, 5 ms. (A) BK current density was larger in Kcnma1N999S/WT neurons (n=16 neurons, 5 mice) compared to Kcnma1WT/WT (n=14 neurons, 4 mice) at ‒30 mV (p=0.0114), ‒20 (p=0.0210), ‒10 (p=0.0426) voltage steps (indicated with *). (B) BK current density was larger in Kcnma1 D434G/D434G neurons (n=12 neurons, 3 mice) compared to Kcnma1WT/WT (n=10 neurons, 4 mice) at density at ‒40 mV (p=0.0112), ‒30 (p=0.0026), ‒20 (p=0.0031), ‒10 (p=0.0038), 0 (p=0.0078), 10 (p=0.0068), 20 (p=0.0071), 30 (p=0.0088) voltage steps (*). Kcnma1D434G/WT mice (n=9 neurons, 3 mice) had higher BK current density compared to Kcnma1WT/WT at ‒30 mV only (†p=0.0321). (C) BK current density was not different in Kcnma1H444Q/WT neurons (n=7 neurons, 2 mice) compared to Kcnma1WT/WT (n=6 neurons, 3 mice).
-
Figure 2—source data 1
Data file for Figure 2A–C.
- https://cdn.elifesciences.org/articles/77953/elife-77953-fig2-data1-v1.xls
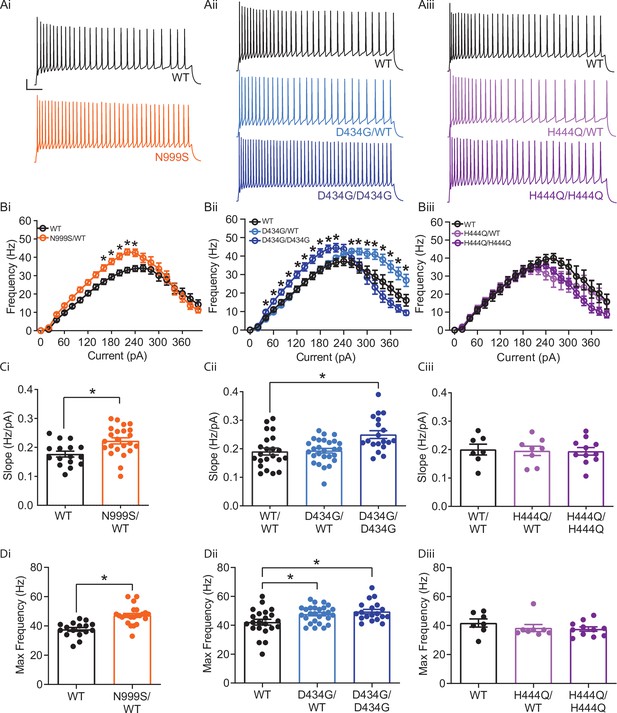
Increased intrinsic excitability in Kcnma1N999S/WT, Kcnma1D434G/WT, and Kcnma1D434G/D434G granule neurons.
In current-clamp mode, step currents from 0 to 400 pA were applied to dentate granule neurons under the same ionic conditions used to record BK currents. (Ai–Aiii) Representative AP trains elicited from the 200 pA current injection step in WT and transgenic neurons. Scale bar: 20 mV, 100 ms. (Bi–Biii) Input-output relationship for firing frequency versus step current injection. Data are presented as mean ± SEM. *p<0.05, two-way repeated measures ANOVA with Bonferroni post hoc. (Bi) Kcnma1N999S/WT (n=23 neurons, 5 mice) firing was higher than Kcnma1WT/WT (n=16 neurons, 5 mice) at 160 pA (p=0.0426), 180 pA (p=0.0143), 200 (p=0.0068), 220 pA (p=0.0009), and 240 pA (p=0.0337) current steps. (Bii) Kcnma1D434G/WT (n=27 neurons, 5 mice) firing was higher than Kcnma1WT/WT (n=22 neurons, 5 mice) at 260 pA (p=0.0452), 280 (p=0.0314), 300 (p=0.0351), 320 (p=0.0177), 340 (p=0.0309), 360 (p=0.0358), 380 (p=0.0312), and 400 (p=0.0444) current steps. Kcnma1D434G/D434G (n=19 neurons, 4 mice) firing was higher than Kcnma1WT/WT at 40 pA (p=0.0266), 60 (p=0.0233), 80 (p=0.0277), 100 (p=0.0130), 120 (p=0.0074), 140 (p=0.0119), 160 (p=0.0084), 180 (p=0.0063), 200 (p=0.0059), and 220 (p=0.0261) current steps. (Biii) Kcnma1H444Q/WT (n=8 neurons, 2 mice) and Kcnma1H444Q/H444Q (n=11 neurons, 2 mice) firing was not different than Kcnma1WT/WT (n=7 neurons, 1 mouse) at any current step (p=0.3222). (Ci–Ciii) Initial slope for the firing rate gain between 0 and 160 pA current injections. Data are presented as mean ± SEM, with individual data points. (Ci) Kcnma1N999S/WT firing slope was increased compared to WT (*p=0.0034; t-test). (Cii) Kcnma1 D434G/D434G firing slope was increased compared to WT (*p=0.0051; one-way ANOVA), Kcnma1 D434G/WT slopes were unchanged (p=0.9774). (Ciii) Kcnma1H444Q/WT and/or Kcnma1H444Q/H444Q firing slopes were not different than WT (p=0.9658). (Di–Diii) Maximum firing frequency. Data are presented as mean ± SEM. (Di) Maximal firing from Kcnma1N999S/WT neurons was increased compared to WT (*p<0.0001; t-test). (Dii) Maximal firing from Kcnma1 D434G/WT and Kcnma1 D434G/D434G neurons was increased compared to WT (*p=0.0387 and p=0.0111, respectively; one-way ANOVA). (Diii) Maximal firing from Kcnma1H444Q/WT and/or Kcnma1H444Q/H444Q neurons was not different than WT (p=0.4625; one-way ANOVA). Passive membrane properties for this dataset appear in Figure 3—figure supplement 1. Action potential waveform analysis for this dataset appears in Figure 3—figure supplement 2.
-
Figure 3—source data 1
Data file for Figure 3B–D.
- https://cdn.elifesciences.org/articles/77953/elife-77953-fig3-data1-v1.xls
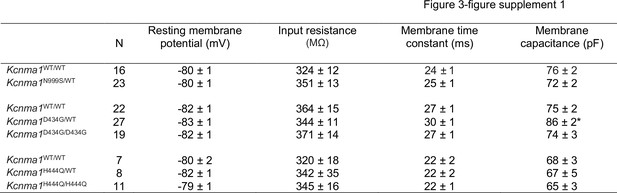
Passive membrane properties.
Data are presented as mean ± SEM. Resting membrane potential (RMP) was ‒80 to ‒82 mV across WT controls. No significant depolarizations were observed between littermate controls and Kcnma1N999S/WT, Kcnma1D434G/D434G, Kcnma1H444Q/WT, and Kcnma1H444Q/H444Q dentate granule neurons (p>0.05, unpaired t-test and one-way ANOVA, respectively). Similarly, the range for input resistance (Ri) was 320–364 MΩ for WT neurons and was not different in any transgenic condition. These results are consistent with previous studies in dentate granule cells finding no effect of BK channel inhibition on RMP or Ri (Bock and Stuart, 2016; Brenner et al., 2005). The only condition showing a difference from the respective WT control was a greater membrane capacitance (Cm) in Kcnma1D434G/WT neurons (*p=0.0036). An explanation for this change in Cm is unclear from the data. However, this difference could have the potential to reduce the firing gain at lower current injections, preventing the Kcnma1D434G/WT input-output curve from looking similar to Kcnma1N999S/WT (Figure 3Bi, Bii).
-
Figure 3—figure supplement 1—source data 1
Data file for Figure 3—figure supplement 1.
- https://cdn.elifesciences.org/articles/77953/elife-77953-fig3-figsupp1-data1-v1.xls
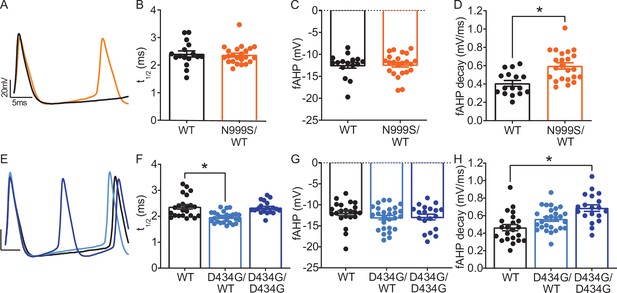
Kcnma1N999S/WT, Kcnma1D434G/WT, and Kcnma1D434G/D434G action potential waveforms.
(A) Superimposed Kcnma1N999S/WT and Kcnma1WT/WT waveforms from the 10th action potential at the 200 pA current injection from data in Figure 3. (B) Action potential half-width (t1/2) was not different in Kcnma1N999S/WT neurons versus WT controls (p=0.6617; Mann-Whitney test). (C) Fast afterhyperpolarizations (fAHP) amplitude was not different in Kcnma1N999S/WT neurons versus WT controls (p=9214; Mann-Whitney test). (D) AHP decay 3 ms after the peak was faster in Kcnma1N999S/WT neurons (Kcnma1N999S/WT 0.60±0.03 mV/ms) compared to WT controls (0.41±0.03 mV/ms, *p=0.0002; t-test). In panels B-D, Kcnma1N999S/WT (n = 23 neurons) and Kcnma1WT/WT (n = 16 neurons). (E) Superimposed Kcnma1D434G/D434G, Kcnma1D434G/WT, and Kcnma1WT/WT waveforms from the 10th action potential at the 200 pA current injection from data in Figure 3. (F) t1/2 was different in Kcnma1D434G/WT (*p=0.0002; Kruskal-Wallis test), but not Kcnma1D434G/D434G neurons (p=0 > 0.9999). (G) fAHP amplitudes were comparable in Kcnma1D434G mice (p=0.3441; Kruskal-Wallis test). (H) AHP decay 3 ms after the peak was faster in Kcnma1D434G/D434G neurons (0.69±0.04 mV/ms) compared to WT controls (0.46±0.04 mV/ms, *p=0.0002), but not in Kcnma1D434G/WT (0.56±0.02 mV/ms, p=0.0620; one-way ANOVA). In panels F-H, Kcnma1D434G/D434G (n = 19 neurons), Kcnma1D434G/WT (n = 27 neurons), and Kcnma1WT/WT (n = 22 neurons). Data are presented as mean ± SEM, with individual data points.
-
Figure 3—figure supplement 2—source data 1
Data file for Figure 3—figure supplement 2B-H.
- https://cdn.elifesciences.org/articles/77953/elife-77953-fig3-figsupp2-data1-v1.xls
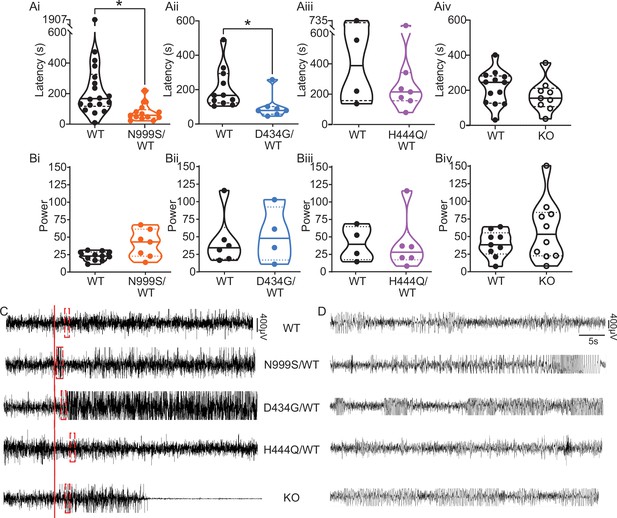
Pentylenetetrazol (PTZ)-induced seizures in mice.
(Ai–Aiv) Latency to initial seizure after PTZ injection. Data are individual mice with median and inter-quartile range. (Ai) Latency was decreased in Kcnma1N999S/WT mice (n=13) compared to Kcnma1WT/WT (n=18, *p=0.0006; Mann-Whitney test). (Aii) Latency was decreased in Kcnma1D434G/WT mice (n=7) compared to Kcnma1WT/WT (n=11, *p=0.0041; Mann-Whitney test). (Aiii) Seizure latency was comparable between Kcnma1H444Q/WT (n=7) and Kcnma1WT/WT (n=4, p=0.5273; Mann-Whitney test). (Aiv) No differences were found in seizure latency between Kcnma1‒/‒ (n=9) and Kcnma1+/+ mice (n=13, p=0.2282; Mann-Whitney test). (Bi–iv) Total EEG power after PTZ injection (y-axis in µV2/Hz × 102). Data are individual mice with median and inter-quartile range. (Bi) EEG power was not different between Kcnma1N999S/WT (n=7) and Kcnma1WT/WT (n=11, p=0.0619; t-test). (Bii) Kcnma1D434G/WT (n=4) was not different from Kcnma1WT/WT (n=6, p=0.7563; t-test). (Biii) Kcnma1H444Q/WT (n=6) was not different from Kcnma1WT/WT (n=4, p=0.9641; t-test). (Biv) Kcnma1‒/‒ (n=10) was not different from Kcnma1+/+ (n=9, p=0.2134; t-test). (C) Representative EEG traces over 45 min at baseline and after PTZ injection (red line). (D) Expanded EEG traces for the first seizure indicated with the red boxes in (C). Representative videos for this dataset appear in Figure 4—videos 1–4.
-
Figure 4—source data 1
Data file for Figure 4A, B.
- https://cdn.elifesciences.org/articles/77953/elife-77953-fig4-data1-v1.xls
Kcnma1WT/WT.
The mouse displays clonic extensions of its hindlimbs accompanied by brief generalized epileptiform discharges on EEG (<2 s bursts) (t = ~20 s). As the video progresses, epileptiform bursts increase in frequency and duration, but the mouse does not develop tonic-clonic seizures. This video represents a typical response for WT animals and illustrates the baseline behavioral changes to a 40 mg/kg pentylenetetrazol (PTZ) dose. Blue channel = left hemisphere, pink channel = right hemisphere, teal channel = EMG.
Kcnma1N999S/WT.
This mouse begins having burst of epileptiform discharges on EEG that are accompanied by clonic extension of the hindlimbs and brief myoclonic jerking movements (t = ~10 s). Discharges become more sharply contoured, increase in frequency, and are generalized at t = ~30 s. Following this (t = ~53 s), the discharges evolve into a generalized tonic-clonic seizure lasting 13 s. After the seizure, the EEG is suppressed with intermittent epileptiform bursts lasting approximately 1 s. Black channel = left hemisphere, teal channel = right hemisphere, purple channel = EMG.
Kcnma1D434G/WT.
The video starts with the mouse in behavioral arrest that progresses to brief tonic-clonic seizures (t = ~30 s). Tonic-clonic seizures increase in duration and continue until the end of the video segment. Between seizures, there is severe EEG suppression (t=55 s). Orange channel = left hemisphere, green channel = right hemisphere, red channel = EMG.
Kcnma1‒/‒.
The video starts with the mouse having bursts of epileptiform discharges without clear behavioral correlates. At t = ~20 s, the mouse has an electrographic seizure with minimal behavioral changes that include myoclonic extension of the hindlimbs and occasional myoclonic jerks. Thereafter, discharges continue on EEG but are accompanied by significant EMG suppression (bottom, red). By t=58 s, electrographic seizures are longer in duration, and bursts of activity are higher in frequency. However, the EMG remains suppressed and the animal is shown in behavioral arrest with myoclonic extension of the hindlimbs. As the seizure progresses (t=90 s), the mouse walks in the cage, but no abnormal tonic-clonic activity is observed. Orange channel = left hemisphere, green channel = right hemisphere, red channel = EMG.
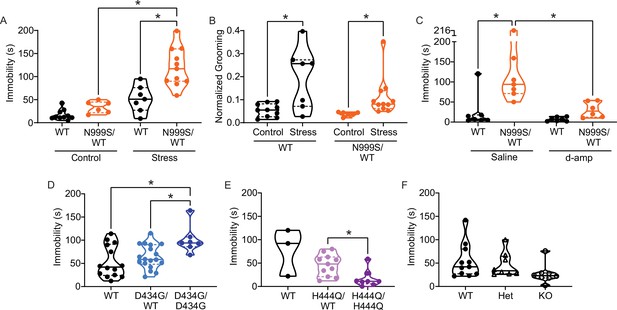
Stress-induced paroxysmal dyskinesia.
(A) Control: Without restraint stress, there was no difference in the time spent immobile between Kcnma1WT/WT (n=10) and Kcnma1N999S/WT mice (n=6, p>0.9999; two-way ANOVA with Bonferroni post hoc). Restraint stress: Immobility time was longer for restrained Kcnma1N999S/WT mice (n=11) compared to Kcnma1WT/WT (n=7, *p=0.0001; one-way ANOVA), and between restrained Kcnma1N999S/WT mice (n=11) compared to unrestrained Kcnma1N999S/WT mice (n=6, *p<0.0001). In contrast, unrestrained Kcnma1WT/WT mice (n=10) had no differences from restrained Kcnma1WT/WT mice (n=7, p=0.1174). (B) Grooming behavior increased in restrained Kcnma1WT/WT mice (n=7) compared to unrestrained Kcnma1WT/WT mice (n=10, *p=0.0300; t-test), and in restrained Kcnma1N999S/WT mice (n=11) compared to unrestrained Kcnma1N999S/WT mice (n=6, *p=0.0174; t-test). (C) Immobility time was longer for saline-treated Kcnma1N999S/WT mice (n=7) compared to Kcnma1WT/WT (n=7, *p=0.0018) and d-amp-treated Kcnma1N999S/WT mice (n=6, *p=0.0053; two-way ANOVA with Bonferroni post hocs). There was no difference between d-amp-treated Kcnma1WT/WT mice (n=7), d-amp-treated Kcnma1N999S/WT mice (n=6, p>0.9999), and saline-treated Kcnma1WT/WT mice (n=7, p>0.9999). (D) After restraint, Kcnma1D434G/D434G mice (n=7) spent more time immobile compared to Kcnma1WT/WT mice (n=14, *p=0.0166; one-way ANOVA). However, Kcnma1D434G/WT mice were not different (n=18, p=0.7174). (E) Immobility time was shorter in restrained Kcnma1H444Q/H444Q mice (n=8) compared to Kcnma1H444Q/WT mice (n=11, *p=0.0081; t-test). Kcnma1WT/WT mice were not included in the statistical analysis due to small sample size (n=3). (F) Kcnma1–/– mice (n=8) had reduced immobility compared to Kcnma1–/+ mice (n=8) and Kcnma1+/+ mice (n=11, p=0.0535; Kruskal-Wallis test). Data are individual mice with median and inter-quartile range. Representative videos for this dataset appear in Figure 5—video 5‒1.
-
Figure 5—source data 1
Data file for Figure 5A–F.
- https://cdn.elifesciences.org/articles/77953/elife-77953-fig5-data1-v1.xls
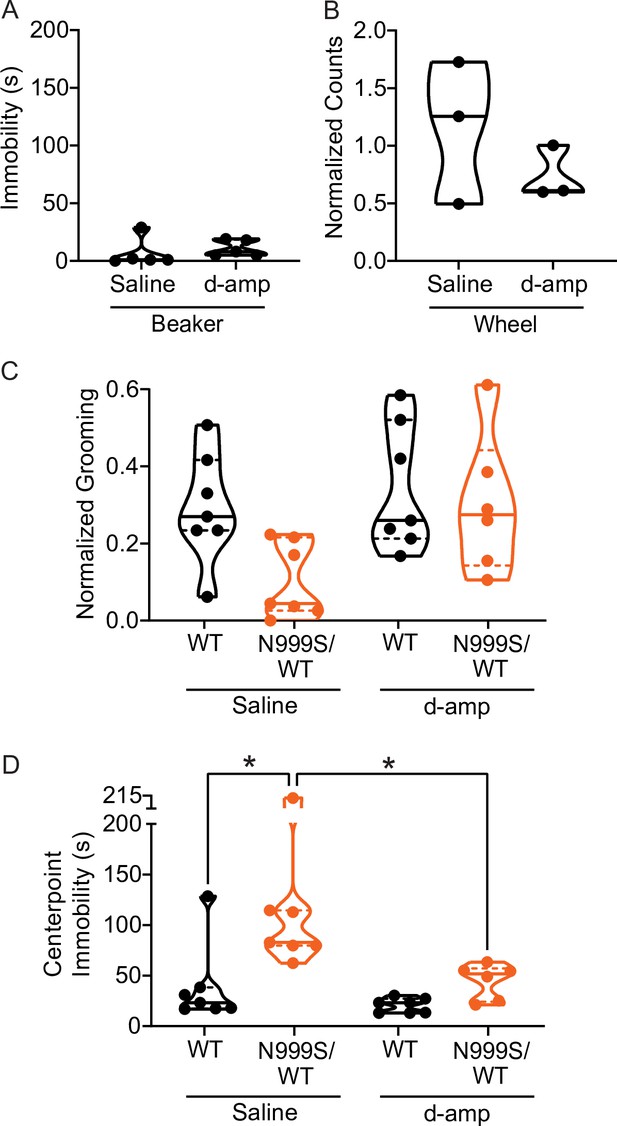
Locomotor, stress, and immobility analysis methodology controls in Kcnma1N999S/WT mice.
(A) Unrestrained WT C57BL6J-background mice were used to test for any baseline effects of the injection procedures (saline) or d-amp (0.5 mg/kg) on immobility in the beaker assay, assessed 30 min after injection. No difference between saline (n=5) and d-amp-injected mice (n=5, p=0.1429, Mann-Whitney test) was observed. (B) Unrestrained WT C57BL6J-background mice were used to test for any baseline effects of d-amp (0.5 mg/kg) on locomotor wheel running activity. Normalized activity after injection was comparable between saline (n=3) and d-amp-injected mice (n=3, p=0.7000, Mann-Whitney test). (C) Kcnma1WT/WT and Kcnma1N999S/WT mice used in the restraint-induced immobility assay in main Figure 5C were scored for grooming behavior after d-amp treatment. No significant differences were present between saline-injected Kcnma1WT/WT mice (n=7), saline-injected Kcnma1N999S/WT mice (n=7, p=0.1456), and d-amp-injected Kcnma1WT/WT mice (n=7, p>0.9999, two-way ANOVA with Bonferroni post hoc). Additionally, d-amp-injected Kcnma1N999S/WT mice (n=6) were not different from d-amp-injected Kcnma1WT/WT mice (n=7, p>0.9999) and saline-injected Kcnma1N999S/WT mice (n=7, p=0.1461). (D) Center point movement parameters were calculated using automated analysis (EthoVision software) from the same videos of restraint stress-induced immobility in main Figure 5C. Immobility time was longer for saline-injected Kcnma1N999S/WT mice (n=7) compared to Kcnma1WT/WT (n=7, *p=0.0077) and d-amp-injected Kcnma1N999S/WT mice (n=6, *p=0.0223; two-way ANOVA with Bonferroni post hoc). In contrast, d-amp-injected Kcnma1N999S/WT mice (n=6) were not different from d-amp-injected Kcnma1WT/WT mice (n=7, p>0.9999) or saline-injected Kcnma1WT/WT mice (n=7, p>0.9999). All data are median and inter-quartile range.
-
Figure 5—figure supplement 1—source data 1
Data file for Figure 5—figure supplement 1A–D.
- https://cdn.elifesciences.org/articles/77953/elife-77953-fig5-figsupp1-data1-v1.xls
Restraint stress-induced dyskinesia.
Five minutes of beaker activity for Kcnma1N999S/WT, Kcnma1WT/WT, and Kcnma1‒/‒ mice after 5 min restraint stress. Video is a representative 1 min segment for each mouse played at 1× speed.
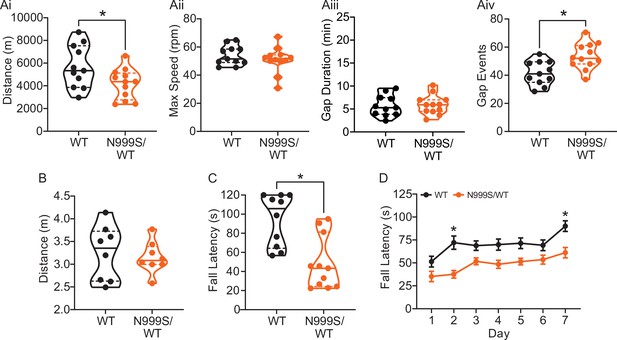
Motor coordination in Kcnma1N999S/WT mice.
(A) Locomotor wheel running parameters calculated from average activity counts over 48 hr from singly housed mice with free access to wheels. (Ai) Distance covered was reduced for Kcnma1N999S/WT (n=12) compared to Kcnma1WT/WT mice (n=11, *p=0.0411; t-test). (Aii) Maximum speed was comparable between Kcnma1N999S/WT (n=11) and Kcnma1WT/WT mice (n=12, p=0.3618; t-test). (Aiii) Duration of time off wheels (gap duration) was comparable between Kcnma1N999S/WT (n=11) and Kcnma1WT/WT mice (n=12, p=0.8281; t-test). (Aiv) Number of times the mouse was off the wheel (gap events) was higher for Kcnma1N999S/WT (n=12) compared to Kcnma1WT/WT mice (n=11, *p=0.0040; t-test). (B) Open field assay. Kcnma1N999S/WT mice (n=8) covered the same distance as Kcnma1WT/WT mice (n=8) in a 15 min trial (p=0.6973; t-test). (C) Acute muscle strength was tested by hanging mice from a stationary platform (cage lid) for 120 s. Fall latency was lower in Kcnma1N999S/WT (n=11) compared to Kcnma1WT/WT mice (n=10, *p=0.0014; Mann-Whitney test) indicating weaker grip strength. (D) Rotarod assay. Fall latency was lower for Kcnma1N999S/WT mice (n=11) on day 2 (*p=0.0045) and day 7 (*p=0.0124) compared to Kcnma1N999S/WT mice (n=12). Motor learning was observable as an improvement in fall latency times across the three trials on each day (data not shown), suggesting the overall impairment was related to motor coordination and not learning. Data are presented as individual data points with median and inter-quartile range (A–C) and mean ± SEM (D). Results for these assays with Kcnma1D434G, Kcnma1H444Q, and Kcnma1–/– mice appear in Figure 6—figure supplements 1 and 2. For these assays, the baseline motor coordination severity fell in the series Kcnma1‒/‒>Kcnma1D434G/D434G > Kcnma1N999S/WT>Kcnma1H444Q/H444Q.
-
Figure 6—source data 1
Data file for Figure 6Ai, Aiv, B–DD.
- https://cdn.elifesciences.org/articles/77953/elife-77953-fig6-data1-v1.xls
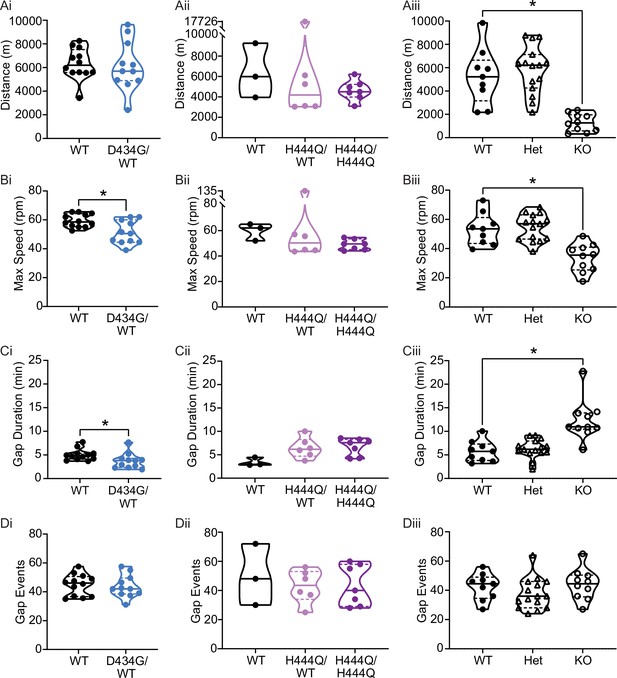
Motor coordination in Kcnma1D434G, Kcnma1H444Q, and Kcnma1‒/‒ mice.
(Ai) Distance was comparable between Kcnma1D434G/WT (n=11) and Kcnma1WT/WT mice (n=12, p=0.8118; t-test). (Aii) Distance was comparable between Kcnma1H444Q/H444Q (n=7) and Kcnma1H444Q/WT (n=6, p=0.4880; t-test). Kcnma1WT/WT mice were not included in statistical analysis in panels Aii–Dii due to small sample size. (Aiii) Distance was reduced for Kcnma1–/– (n=10) compared Kcnma1+/+ (n=9, *p=0.0032), but not for Kcnma1–/+ mice (n=15, p=0.9057; one-way ANOVA). (Bi) Maximum speed was lower for Kcnma1D434G/WT (n=11) compared to Kcnma1WT/WT mice (n=12, *p=0.0085; t-test). (Bii) Maximum speed was comparable between Kcnma1H444Q/H444Q (n=7) and Kcnma1H444Q/WT (n=6, p=0.3634; t-test). (Biii) Maximum speed was lower for Kcnma1–/– (n=10) compared to Kcnma1+/+ (n=9, *p=0.0024), but not for Kcnma1–/+ mice (n=15, p=0.9871; one-way ANOVA). (Ci) Gap duration was reduced for Kcnma1D434G/WT (n=11) compared to Kcnma1WT/WT mice (n=12, *p=0.0467; t-test). (Cii) Gap duration was comparable between Kcnma1H444Q/H444Q (n=7) and Kcnma1H444Q/WT mice (n=6, p=0.8326; t-test). (Ciii) Gap duration was higher in Kcnma1–/– (n=10) compared to Kcnma1+/+ mice (n=9, *p=0.0026), but not for Kcnma1–/+ mice (n=15, p=0.8987; one-way ANOVA). (Di) Gap events were comparable between Kcnma1D434G/WT (n=11) and Kcnma1WT/WT mice (n=12, p=0.7425; t-test). (Dii) Gap events were comparable between Kcnma1H444Q/H444Q (n=7) and Kcnma1H444Q/WT (n=6, p=0.9341; t-test). (Diii) Gap events were comparable for Kcnma1–/– (n=10), Kcnma1–/+ (n=15), and Kcnma1+/+ mice (n=9, p=0.3047; one-way ANOVA). All data are presented as individual data points with median and inter-quartile range.
-
Figure 6—figure supplement 1—source data 1
Data file for Figure 6—figure supplement 1Ai, Di, Aii–Dii, Aiii–Diiii.
- https://cdn.elifesciences.org/articles/77953/elife-77953-fig6-figsupp1-data1-v1.xls
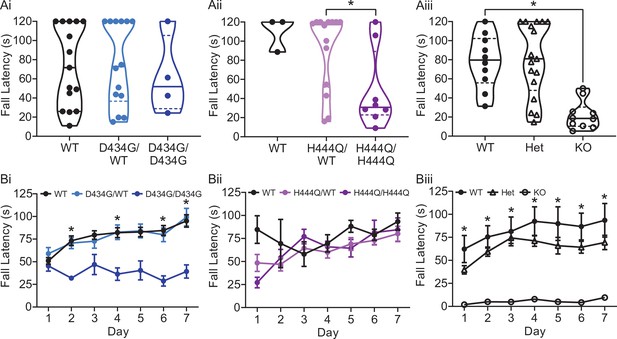
Hanging wire and rotarod in Kcnma1D434G, Kcnma1H444Q, and Kcnma1‒/‒ mice.
(Ai-iii) Hanging wire assay. Data are presented as individual data points with median and inter-quartile range. (Ai) Fall latency was comparable for Kcnma1D434G/D434G (n=4), Kcnma1D434G/WT (n=11), and Kcnma1WT/WT mice (n=11, p=0.8329; Kruskal-Wallis test), providing no evidence for acute differences in strength. (Aii) Fall latency was reduced for Kcnma1H444Q/H444Q (n=8) compared to Kcnma1H444Q/WT mice (n=14, *p=0.0465; Mann-Whitney test). Kcnma1WT/WT mice were not included in the statistical analysis due to small sample size (n=3). (Aiii) Fall latency times were lower for Kcnma1‒/‒ mice (n=10) compared to Kcnma1+/+ mice (n=10, *p=0.0036), but not for Kcnma1‒/+ mice (n=17, p>0.9999; Kruskal-Wallis test). No Kcnma1‒/‒ mouse had the ability to hang on longer than 60 s, consistent with previous reports (Meredith et al., 2004; Sausbier et al., 2004; Wang et al., 2020; Yao et al., 2021). (Bi-iii) Time to fall in rotarod assay. Data are presented as mean ± SEM. (Bi) Fall latency was lower for Kcnma1D434G/D434G mice (n=4) on day 2 (p<0.0001), day 4 (p=0.0030), day 6 (p<0.0001), and day 7 (p=0.0009) compared to Kcnma1WT/WT mice (n=21), but were comparable in Kcnma1D434G/WT mice (n=18; *p<0.05, repeated measures ANOVA with Bonferroni post hoc). (Bii) Fall latency was not different between Kcnma1H444Q/H444Q (n=7) and Kcnma1H444Q/WT (n=10; repeated measures ANOVA with Bonferroni post hoc); however, performance was highly variable, reducing the ability to make a firm conclusion from this data. Kcnma1WT/WT mice were not included in the statistical analysis due to small sample size (n=2). (Biii) Fall latency was lower for Kcnma1‒/‒ mice (n=6) on day 1 (p=0.0277), day 2 (p=0.0056), day 3 (p=0.0122), day 4 (p=0.0081), day 5 (p=0.0166), day 6 (p=0.0071), and day 7 (p=0.0168) compared to Kcnma1+/+ mice (n=6), but were comparable in Kcnma1‒/+ mice (n=13; *p<0.05, repeated measures ANOVA with Bonferroni post hoc).
-
Figure 6—figure supplement 2—source data 1
Data file for Figure 6—figure supplement 2Ai–Aiii, Bi–Biiii.
- https://cdn.elifesciences.org/articles/77953/elife-77953-fig6-figsupp2-data1-v1.xls
Tables
Reagent type (species) or resource | Designation | Source or reference | Identifiers | Additional information |
---|---|---|---|---|
Recombinant DNA reagent | BKN999S | This paper | MG279689 with rs886039469 | BK channel expression construct in pcDNA3.1+ (Figure 1) |
Recombinant DNA reagent | BKD434G | This paper | MG279689 with rs137853333 | BK channel expression construct in pcDNA3.1+ (Figure 1) |
Recombinant DNA reagent | BKH444Q | This paper | MG279689 with c.1332C>G, p.H444Q | BK channel expression construct in pcDNA3.1+ (Figure 1) |
Recombinant DNA reagent | BKWT | Genbank | hBKQEERL MG279689 | BK channel expression construct in pcDNA3.1+ (Figure 1) |
Cell line (Homo sapiens) | HEK293T | ATCC | CRL-11268 | |
Chemical compound, drug | DMEM | Gibco, Life Technologies Corp | Cat. #11995-065 | |
Chemical compound, drug | Fetal bovine serum | Sigma-Aldrich | Cat. #4135 | |
Chemical compound, drug | Penicillin/streptomycin | Mediatech Inc | Cat. #30-002 Cl | |
Chemical compound, drug | L-glutamine | Mediatech Inc | Cat. #25-005 Cl | |
Chemical compound, drug | Trans-IT LT1 | Mirius Biological | ||
Chemical compound, drug | Poly-L-lysine | Sigma-Aldrich | Cat. #P4832 | |
Chemical compound, drug | Dextroamphetamine sulfate | Sigma-Aldrich | Cat. #1180004 | |
Gene (Mus musculus) | Kcnma1 | Gene Bank; Ensembl | ID: 16531; ENSMUSG00000063142 | |
Strain (Mus musculus) | C57BL/6J | Jackson Laboratories | Stock #000664 | |
Genetic reagent (Mus musculus) | Kcnma1N999S | This paper | Gene ID:16531 with rs886039469 | Mouse line maintained in A. Meredith’s lab (Figure 1—figure supplement 1) |
Genetic reagent (Mus musculus) | Kcnma1D434G | This paper | Gene ID:16531 with rs137853333 | Mouse line maintained in A. Meredith’s lab (Figure 1—figure supplement 1) |
Genetic reagent (Mus musculus) | Kcnma1H444Q | This paper | Gene ID:16531 with c.1332C>G, p.H444Q | Mouse line maintained in A. Meredith’s lab (Figure 1—figure supplement 1) |
Sequence-based reagent (oligonucleotides) | N999S gRNA | Integrated DNA Technologies | CTGTATGAAGT TACTGTTAT | |
Sequence-based reagent (oligonucleotides) | D434G/H444Q gRNA | Integrated DNA Technologies | GGACCGGGATGA TGTCAACG | |
Sequence-based reagent (oligonucleotides) | N999S donor | Integrated DNA Technologies | AGATACTAAGAAAA GTTGTAATTTGGAC ATCAATTGTGATTTT CGGTGTTGGCTTAA GAATGCTTCTCTTC TACCTTCTTT CTCC AGACATAtTTC AgTGACAATATtCTCA CCCTAATACGGACCC TGGTGACAGGAGGAG CCACACCA | |
Sequence-based reagent (oligonucleotides) | D434G donor | Integrated DNA Technologies | CTCTGGAGAGTGTCT CTAACTTCCTGAAGG ACTTTCTGCACAAGG ACCGtGgTGATGTCAA CGTtGAGATTGTCTTT CTTCACAAGTAAGAGC CCCCTGCTGCCACCA GACCCTGCCACC | |
Sequence-based reagent (oligonucleotides) | H444Q donor | Integrated DNA Technologies | CTCAGAGAGAAGCAT GAGTTTAGGTGGCAG GGTCTGGTGGCAGCA GGGGGCTCTTACTTcT GcAGAAAGACgAT CTCgACGTTGACATC ATCCCGGTCCTTGTG CAGAAAGTCCTTCAGG | |
Sequence-based reagent (oligonucleotides) | N999S genotyping primer (F) | Transnetyx, Inc | TCGGTGTTGGCTTA AGAATGCTT | Kcnma1N999S |
Sequence-based reagent (oligonucleotides) | N999S genotyping primer (R) | Transnetyx, Inc | CCTCAGCTATTAGAG CCTCGAGCTC | Kcnma1N999S |
Sequence-based reagent (oligonucleotides) | WT genotyping reporter | Transnetyx, Inc | CAGACATACTTCAAT GACAATAT | Kcnma1N999S |
Sequence-based reagent (oligonucleotides) | N999S genotyping reporter | Transnetyx, Inc | CAGACATATTTCAGT GACAATAT | Kcnma1N999S |
Sequence-based reagent (oligonucleotides) | D434G genotyping primer (F) | Transnetyx, Inc | CTCTAACTTCCTGAA GGACTTTCTGCACA | Kcnma1D434G |
Sequence-based reagent (oligonucleotides) | D434G genotyping primer (R) | Transnetyx, Inc | CAGAGAGAAGCATG AGTTTAGGTGGCA | Kcnma1D434G |
Sequence-based reagent (oligonucleotides) | WT genotyping reporter | Transnetyx, Inc | ACCGGGATGATGTCA | Kcnma1D434G |
Sequence-based reagent (oligonucleotides) | D434G genotyping reporter | Transnetyx, Inc | ACCGTGGTGATGTCAA | Kcnma1D434G |
Sequence-based reagent (oligonucleotides) | H444Q genotyping primer (F) | Transnetyx, Inc | CTGTGGACACATTAC TCTGGAGAGTG | Kcnma1H444Q |
Sequence-based reagent (oligonucleotides) | H444Q genotyping primer (R) | Transnetyx, Inc | GGGTCTGGTGGCAGCA | Kcnma1H444Q |
Sequence-based reagent (oligonucleotides) | WT genotyping reporter | Transnetyx, Inc | TCTTACTTGTGAAGAAAG | Kcnma1H444Q |
Sequence-based reagent (oligonucleotides) | H444Q genotyping reporter | Transnetyx, Inc | CTCTTACTTCTGCAGAAAG | Kcnma1H444Q |
Genetic reagent (Mus musculus) | Kcnma1‒/+ | PMID:15184377 DOI: 10.1074/jbc.M405621200 or available from the Jackson Laboratories | Slo1‒/‒ (Meredith Lab) or Stock #035902 (B6.129(FVB)-Kcnma1tm1Rwa/J, Jackson Laboratories) | Breeder to generate Kcnma1‒/‒ |
Sequence-based reagent (oligonucleotides) | WT genotyping primer (F) | Transnetyx, Inc | CATCATACCGGTGACCATGGA | Kcnma1‒/‒ |
Sequence-based reagent (oligonucleotides) | WT genotyping primer (R) | Transnetyx, Inc | CCAAGAAAGCCCACCACATG | Kcnma1‒/‒ |
Sequence-based reagent (oligonucleotides) | WT genotyping reporter | Transnetyx, Inc | CCCGGCTGTCGCACG | Kcnma1‒/‒ |
Sequence-based reagent (oligonucleotides) | Neomycin genotyping primer (F) | Transnetyx, Inc | GGGCGCCCGGTTCTT | Kcnma1‒/‒ |
Sequence-based reagent (oligonucleotides) | Neomycin genotyping reporter | Transnetyx, Inc | CCTCGTCCTGCAGTTCATTCA | Kcnma1‒/‒ |
Sequence-based reagent (oligonucleotides) | Neomycin genotyping primer (R) | Transnetyx, Inc | ACCTGTCCGGTGCCC | Kcnma1‒/‒ |
Commercial assay, kit | miRNeasy Mini Kit | Qiagen | Cat. #217004 | |
Commercial assay, kit | Mouse Clariom D Assay | Applied Biosystems | Cat. #902514 | |
Chemical compound, drug | Paxilline | alomone labs | Cat. #P-450 | |
Chemical compound, drug | 4-Aminopyridine | Sigma-Aldrich | Cat. #275875 | |
Chemical compound, drug | TTX | alomone labs | Cat. #T-550 | |
Chemical compound, drug | Pentylenetetrazol | Sigma-Aldrich | Cat. #P6500 | |
Software, algorithm | pClamp10.7 | Molecular Devices | ||
Software, algorithm | Transcriptome Analysis Console Software | ThermoFisher Scientific | TAC version 4.0.1 | |
Software, algorithm | Sirenia Acquisition software | Pinnacle Technology Inc | Version 2.2.4 | |
Software, algorithm | Sirenia Seizure Pro software | Pinnacle Technology Inc | Cat. #9037 | |
Software, algorithm | Prism Software | GraphPad (Dotmatics) | Prism version 9.02 | |
Software, algorithm | Ethovision Software | Noldus Information Technology | Ethovision XT version 11.5 |
Additional files
-
Transparent reporting form
- https://cdn.elifesciences.org/articles/77953/elife-77953-transrepform1-v1.pdf
-
Source code 1
Locomotor wheel activity script.
Python code to calculate wheel speed and the number and duration of activity gaps.
- https://cdn.elifesciences.org/articles/77953/elife-77953-code1-v1.zip