Inhibition of SHP-1 activity by PKC-θ regulates NK cell activation threshold and cytotoxicity
Abstract
Natural killer (NK) cells play a crucial role in immunity, killing virally infected and cancerous cells. The balance of signals initiated upon engagement of activating and inhibitory NK receptors with cognate ligands determines killing or tolerance. Nevertheless, the molecular mechanisms regulating rapid NK cell discrimination between healthy and malignant cells in a heterogeneous tissue environment are incompletely understood. The SHP-1 tyrosine phosphatase is the central negative NK cell regulator that dephosphorylates key activating signaling proteins. Though the mechanism by which SHP-1 mediates NK cell inhibition has been partially elucidated, the pathways by which SHP-1 is itself regulated remain unclear. Here, we show that phosphorylation of SHP-1 in NK cells on the S591 residue by PKC-θ promotes the inhibited SHP-1 ‘folded’ state. Silencing PKC-θ maintains SHP-1 in the active conformation, reduces NK cell activation and cytotoxicity, and promotes tumor progression in vivo. This study reveals a molecular pathway that sustains the NK cell activation threshold through suppression of SHP-1 activity.
Editor's evaluation
The study by Dr. Barda-Saad and colleagues deals with natural killer (NK) cells activation that depends on a balance between stimulatory and inhibitory signals. Inhibition of NK cells results from the recruitment of the protein tyrosine phosphatase, SHP-1, which dephosphorylates vital signaling molecules and thus reverses NK cell activation. This study describes how PKC regulates the activity of SHP-1 in the early stages of activation and inhibition of NK cell synapses and, by doing so, regulates their adaptation to the microenvironment in which they act.
https://doi.org/10.7554/eLife.73282.sa0Introduction
Natural killer (NK) cells are a class of lymphocytes that comprise a central component of the innate immune system. They patrol the body and kill virally transformed and cancerous cells. NK cell effector functions are carried out by the release of cytotoxic granules containing perforin and Granzyme B, which induce target cell death (Pipkin and Lieberman, 2007; Topham and Hewitt, 2009). Furthermore, NK cells produce cytokines and chemokines, such as IFN-γ, which activate the adaptive immune response (Malhotra and Shanker, 2011).
Activation or inhibition of NK function was shown to depend on the balance between signals derived from activating and inhibitory receptors on the NK cell surface (Lanier, 2005). Various motifs on the cytoplasmic tails of these receptors were shown to induce distinct downstream signaling cascades. These include immunoreceptor tyrosine-based activation motifs (ITAMs) found on cytoplasmic tails of the transmembrane adaptor proteins CD3ζ, FcɛRIγ, and DAP12, or the YINM motif on the DAP10 adaptor, which associates with the NKG2D receptor (André et al., 2004). Another group of motifs includes the immunoreceptor tyrosine-based switch motifs (ITSMs) on the CD244 receptor (Vyas et al., 2002b; Lanier, 2003; Feng et al., 2005). Though NK cell receptors are well characterized, the molecular mechanisms downstream to receptor ligation, and how these are integrated to achieve the appropriate activation state, are incompletely understood.
Inhibitory NK cell receptors express a separate target sequence for signal suppression, consisting of immunoreceptor tyrosine-based inhibition motifs (ITIMs) expressed on the cytoplasmic tails (Long, 2008). The key receptors facilitating NK inhibition recognize major histocompatibility class 1 (MHC-I) molecules expressed on the majority of cells in the body. These receptors include the killer cell immunoglobulin-like receptor (KIR) family in humans and primates, the Ly49 family in mice, and NKG2A/CD94 receptors expressed in both primates and mice (Orr and Lanier, 2010). NGK2A/CD94 were shown to have specificity for the human leukocyte antigen (HLA) isoform E (HLA-E), whereas the KIR family of inhibitory receptors was shown to associate with HLA-C and HLA-B (Moretta et al., 1994; Wagtmann et al., 1995). Engagement of these receptors with their cognate ligands and phosphorylation of their ITIM motifs recruits the Src-homology 2-domain (SH2)-containing protein tyrosine phosphatase (PTP), SHP-1. SHP-1 consequently dephosphorylates key proteins involved in the activating signaling pathway, thereby downregulating NK cell activation (Stebbins et al., 2003; Matalon et al., 2016). SHP-1 comprises a catalytic phosphatase domain near its C′ terminus, two phospho-tyrosine binding SH2 domains toward its N′ terminus, and sites of tyrosine and serine phosphorylation at the end of its C′-terminal tail (Lorenz, 2009). In its autoinhibited closed state, the SHP-1 N′ SH2 domain associates with the catalytic domain, blocking access to its substrates.
Binding of SHP-1 SH2 domains to phosphorylated tyrosine residues on the ITIM of the inhibitory receptors induces a conformational alteration in SHP-1. This releases it from the inhibited state and enables its catalytic activity, that is, dephosphorylation of key signaling molecules and inhibition of NK cell function (Wang et al., 2011). SHP-1 substrates in NK cells include the guanine nucleotide exchange factor (GEF), VAV1 (Stebbins et al., 2003), phospholipase C gamma 1/2 (PLCγ1/2), and linker for activation of T cells (LAT), recently identified by our group (Matalon et al., 2016).
Though SHP-1 function is critical for correct NK cell immune responses, the manner by which its catalytic activity is regulated remains unclear. Elucidating this mechanism is especially important since NK cells engage target cells expressing both activating and inhibitory receptors, and SHP-1 recruitment to ITIMs decreases the activity of NK cells toward potential targets, despite expression of activating ligands. Therefore, upregulation of ligands for inhibitory NK receptors that express ITIMs by cancer cells, such as HLA-E and PD-L1, may repress NK cell activity through SHP-1 recruitment and activity (Carretero et al., 1998; Chemnitz et al., 2004). Identifying the factors that regulate SHP-1 activity may lead to a better understanding of how NK cell activity is accurately and rapidly tuned in tissues containing both healthy and transformed cells. We recently revealed that actin retrograde flow (ARF) induces a physical conformational change in the SHP-1 molecule to facilitate its activity (Matalon et al., 2018). However, key questions remain unresolved. SHP-1 was shown to localize to the lytic NK cell immunological synapse (NKIS) (Vyas et al., 2002a), yet the mechanism by which its activity at the lytic synapse is regulated was not determined. Furthermore, it is not clear how termination of SHP-1 activity occurs after NK cells are successfully inhibited, thereby enabling NK cells to function in subsequent interactions with activating target cells.
Thus, since SHP-1 is a critical checkpoint molecule in NK cells and regulates the NK cell activation threshold, it is of great interest to understand the underlying mechanisms that control its catalytic activity during the NK cell response.
Here, we demonstrate that in NK cells SHP-1 is heavily phosphorylated on the serine 591 residue (S591) via Protein kinase c theta (PKC-θ) (and hence dormant) during activating but not inhibitory responses, within the first 5 min of NK cell activation. This phosphorylation is dynamic and increases along the progression of the inhibitory NKIS, while slightly decreasing during progression of the activating NKIS. SHP-1 phosphorylation dynamics were also closely correlated with changes in SHP-1 catalytic conformations. Blocking PKC-θ-mediated phosphorylation of SHP-1 restored SHP-1 activity and inhibited NK cell activation. Furthermore, NK cells bearing a phosphor-mimetic point mutation that suppresses SHP-1 activity 1 at the serine 591 residue were highly activated. Finally, knockout of SHP-1 in NK cells rescued PKC-θ silencing and promoted tumor clearance in vivo. The PKC-θ-mediated regulation of SHP-1 potentially serves to maintain and prime NK cells in a complex cellular environment consisting of both healthy and malignant cells, requiring finely tuned localized NK cell activity.
Results
SHP-1 S591 phosphorylation in NK cells is a dynamic process, differentially regulated during inhibitory and activating interactions
It remains unclear how SHP-1 activity is regulated throughout the duration of the NK cell response and how this regulation is maintained in different states, that is, during NK cell activation and inhibition. Furthermore, SHP-1 is recruited to both cytolytic and non-cytolytic NK synapses, demonstrating that different modes of regulation are needed to ensure proper NK cell responses (Vyas et al., 2001; Vyas et al., 2002a). Phosphorylation of SHP-1 was previously shown in different cellular systems (Li et al., 1995; Jones et al., 2004; Liu et al., 2007). The outcome of these molecular processes, however, demonstrated contradictory results, and the molecular regulation of SHP-1 in NK cells has not been fully addressed. We recently employed a mutant YTS-2DL1 knock-in line expressing the SHP-1 phosphor-mimetic serine to aspartic acid residue substitution (SHP-1 S591D), which exhibits increased antitumor NK function relative to WT SHP-1-expressing YTS cells (Ben-Shmuel et al., 2021). To dissect the possible effect of SHP-1 phosphorylation on NK cell function, physiological activating and inhibiting interactions were induced with 721.221 target cells, and S591 phosphorylation patterns were examined in YTS-2DL1 cells and isolated primary NK cells expressing KIR2DL1+ (referred to as pNK-2DL1) from healthy human donors. We conducted functional assays as previously described (Matalon et al., 2016; Matalon et al., 2018) by incubating YTS-2DL1 or pNK-2DL1 with 721.221 target cells either expressing the KIR2DL1 cognate ligand, HLA Cw4 (721-Cw4, which inhibits NK activity), or an irrelevant HLA Cw7 ligand (721-Cw7 or 721-HLA-negative cells [Münz et al., 1997], which promote NK cell activation). Cell lysates were immunoblotted (IB) with anti-pSHP-1 S591 antibody. Our data revealed different SHP-1 phosphorylation profiles during NK cell inhibition and activation after 5 min of incubation (Figure 1A). High S591 phosphorylation levels could be seen during activating interactions (3.06 ± 0.31, p=0.0104), whereas lower SHP-1 S591 phosphorylation was observed during induced inhibitory interactions. The same pattern was observed during incubation of pNK-2DL1 cells with activating 721-HLA-negative cells or with inhibiting 721-Cw4 targets cells (2.51 ± 0.19, p=0.006, Figure 1B). The formation of the immunological synapse (IS) is highly dynamic, involving movement, activation, and termination of signaling complexes (Burroughs and Wülfing, 2002). Therefore, we wished to analyze the change in SHP-1 S591 phosphorylation over time. YTS-2DL1 or pNK-2DL1 cells were incubated with activating or inhibiting 721.221 targets for 5, 10, 15, and 20 min. Strikingly, we found that pS591 on SHP-1 was dramatically altered during formation of the inhibitory NKIS, showing almost no initial phosphorylation after 5 min of incubation, and displaying high phosphorylation by 20 min. Activating NK cell interactions, however, displayed higher SHP-1 S591 phosphorylation during the first 5 min of activation, remaining relatively stable, with a slight (nonsignificant) reduction after 20 min of activation (Figure 1C, Figure 1—figure supplement 1). Collectively, these results suggest that SHP-1 S591 phosphorylation may play a role during NK cell activation and during late inhibitory interactions. This mechanism may attenuate SHP-1 functionality in order to enable NK cell activation within these time frames.
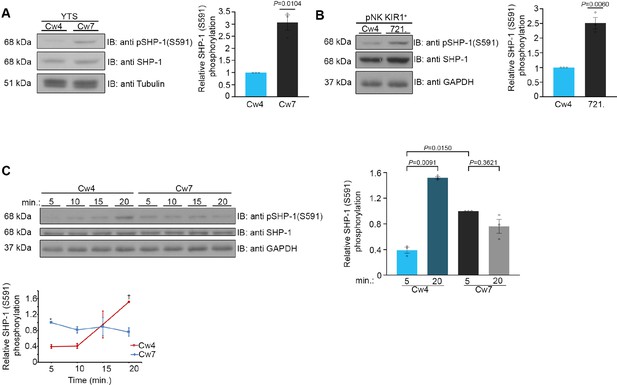
Phosphorylation kinetics of SHP-1 S591 during activating and inhibitory NK cell interactions.
(A) YTS-2DL1 NK cells were incubated with either inhibitory 721-Cw4 HLA or activating 721-Cw7 HLA target cells at 37°C for 5 min, and then lysed. Lysates were separated on SDS-PAGE and immunoblotted with anti-pSHP-1 S591 antibody. SHP-1 S591 phosphorylation levels were measured by densitometric analysis, relative to β-tubulin loading control using ImageJ. Samples were normalized to the YTS-2DL1 sample incubated with 721-Cw4 target after 5 min of activation (p=0.0104, quantification on the right showing the average of three independent experiments). (B) pNK-2DL1 cells were incubated with either 721-Cw4 HLA or 721-HLA-negative target cells at 37°C for 5 min. pSHP-1 S591 levels were determined as in (A) (p=0.0060, quantification on the right showing the average of three independent experiments). (C) YTS-2DL1 cells were incubated with target cells as described in (A), for four different time points, as indicated. pSHP-1 S591 levels were quantitated as in (A). Statistical significance between Cw4 and Cw7 after 5 min of activation (p=0.015), statistical significance between Cw4 at 5 min versus 20 min (p=0.0091). pSHP-1 S591 levels of YTS-2DL1 cells incubated with targets for 5 and 20 min are shown in the bar graph (quantification showing the average of three independent experiments). Data are shown as mean ± SEM. One-sample t-tests (A, B) or one-way ANOVA with Tukey test (C) was used to calculate p-values.
-
Figure 1—source data 1
Representative blots.
- https://cdn.elifesciences.org/articles/73282/elife-73282-fig1-data1-v1.pdf
-
Figure 1—source data 2
Numerical data for all the graphical presentations in Figure 1.
- https://cdn.elifesciences.org/articles/73282/elife-73282-fig1-data2-v1.xlsx
SHP-1 conformational kinetics parallel S591 phosphorylation patterns
To elucidate whether SHP-1 phosphorylation on the S591 residue complements SHP-1 conformation and activation status, an SHP-1 Förster resonance energy transfer (FRET) biosensor construct was cloned into YTS-2DL1 cells as we previously described (Matalon et al., 2018). The FRET sensor was constructed with SHP-1 tagged on the N′ and C′ termini with YFP and CFP, respectively (YFP-SHP-1-CFP). It is known that SHP-1 activation status is correlated with its conformation (Poole and Jones, 2005; Wang et al., 2011). In the closed conformation, the N′ SH2 domain masks the catalytic domain rendering the enzyme inactive, whereas when the catalytic domain is free of the N′ SH2 domain, the protein remains in an open active conformation (Wang et al., 2011). Hence, an inactive SHP-1 protein provides high FRET efficiency due to YFP and CFP proximity, and an active SHP-1 protein demonstrates low FRET efficiency as SHP-1 is in an open conformation, distancing the two reporter proteins. With this construct, we could image the dynamic activation and inhibition status of SHP-1 throughout the lifetime of the NKIS as previously reported (Matalon et al., 2018). YTS-2DL1 cells were transfected with the YFP-SHP-1-CFP FRET sensor construct and incubated with inhibiting 721-Cw4 or activating 721-Cw7 cells stably expressing mCherry in order to distinguish NK and target cells. FRET efficiency was measured using high-resolution microscopy, as previously described (Barda-Saad et al., 2005; Pauker et al., 2012; Fried et al., 2014). In this set of experiments, we chose to focus on very early (5 min) and late (20 min) stages of NK cell: target cell interactions, as they showed the largest change in SHP-1 S591 phosphorylation (Figure 1, Figure 1—figure supplement 1). FRET measurements demonstrated patterns similar to SHP-1 S591 phosphorylation profiles; during initial inhibitory NK:721-Cw4 interactions (5 min), SHP-1 in NK cells displayed low synaptic FRET efficiency, indicating an open and active state (8.62% ± 1.8%), shifting to high FRET efficiency after 20 min of synapse maturation (15.13% ± 1.9%, p=0.0300; Figure 2A and B, top two panels), indicating a closed and inhibited state. During activating interactions, however, SHP-1 in NK cells displayed highest synaptic FRET efficiency after 5 min (18.45% ± 1.9%, p=0.0003 comparing 5 min of activation to inhibition), indicating a closed and inhibited state, while after 20 min of activation, a decrease was observed in SHP-1 FRET efficiency to similar levels as NK:721-Cw4 interactions after 5 min (8.15% ± 1.9%, p=0.006; Figure 2A and B, bottom two panels), indicating an open and active SHP-1 conformation (Matalon et al., 2018). These data demonstrate that SHP-1 conformation, and accordingly, its activation status, changes differentially during activating and inhibiting interactions as the NKIS matures, correlating with S591 phosphorylation patterns. Our results suggest that SHP-1 conformation and catalytic activation are regulated in a temporal manner at both the activating and inhibitory NKIS, and suggest a possible role for SHP-1 S591 phosphorylation on SHP-1 activity in NK cells.
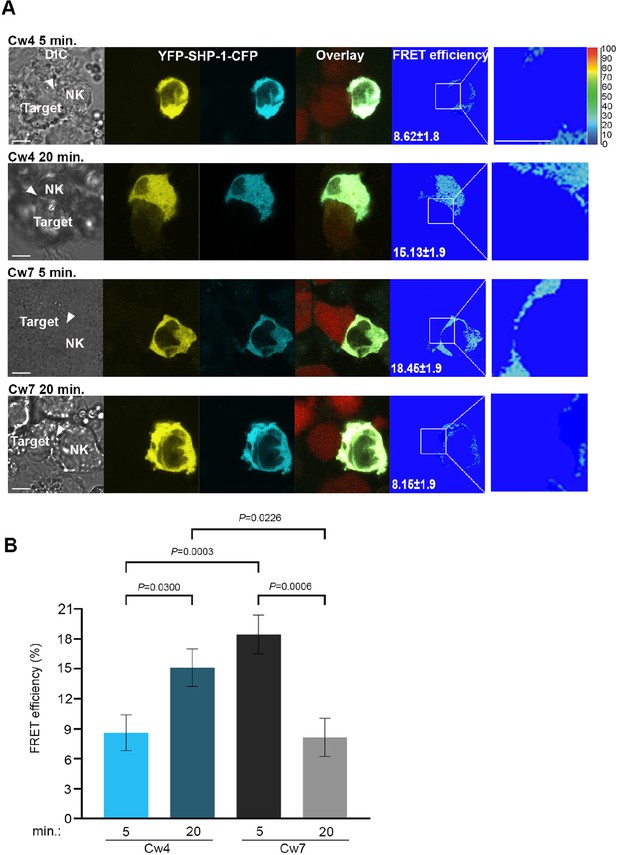
SHP-1 conformational dynamics reflect S591 phosphorylation during activating and inhibitory NK cell interactions.
(A) YTS-2DL1 YFP-SHP-1-CFP cells were incubated over slides pre-seeded with 721-Cw4 (top panels) or Cw7 (bottom panels) target cells expressing mCherry. The cells were incubated for 5 or 20 min at 37°C to enable conjugate formation, and fixed. FRET analysis was performed as indicated. (B) Graph summarizing FRET efficiency following 5 or 20 min activation with Cw4 or Cw7 target cells. For Cw4, 5 and 20 min activation, n = 72 and 62 cell conjugates analyzed, respectively. For Cw7, 5 and 20 min activation, n = 73 and 47 cell conjugates analyzed from three independent experiments, respectively. Data are shown as mean ± SEM. Two-way ANOVA with Tukey test (B) was used to calculate p-values.
-
Figure 2—source data 1
Numerical data for all the graphical presentations in Figure 2.
- https://cdn.elifesciences.org/articles/73282/elife-73282-fig2-data1-v1.xlsx
pSHP-1 distinguishes between NK cell-activating and -inhibiting synapses, and correlates with PKC-θ accumulation at the NKIS
In transformed tissues, NK cells interact with both susceptible targets and bystander cells, which may both influence NK cell function (Zhou et al., 2017). NK cells are capable of serial cytotoxicity and rapidly engage with and detach from these different targets (Choi and Mitchison, 2013; Forslund et al., 2015; Guldevall et al., 2016). It was recently demonstrated that NKIS maintenance is highly regulated, and signaling events that lead to NK cell attachment to new target cells accelerate the detachment from previous targets (Netter et al., 2017; Srpan et al., 2018). Furthermore, highly regulated lytic granule convergence to the microtubule organizing center (MTOC) is crucial for NK cells to avoid bystander cell killing (Hsu et al., 2016). Thus, it is evident that controlled and coordinated signaling events are critical for NK cell target identification and subsequent precise function.
We hypothesized that SHP-1 S591 phosphorylation may thus enable rapid discrimination by NK cells between healthy and malignant targets, facilitating controlled and sequential killing in a heterogeneous environment. To examine whether SHP-1 phosphorylation in NK cells is coordinately directed when NK cells are challenged simultaneously with activating and inhibitory signals, pNK-2DL1 cells were concurrently incubated with both activating K562-CFP and inhibiting 721-Cw4-mCherry stably labeled cells. Synapse intensity of pSHP-1 S591 was assessed in NK cells that were found forming simultaneous dual synapses with both activating and inhibiting cells. Strikingly, NK cells were able to rapidly distinguish between activating and inhibitory targets cells as measured by higher pSHP-1 S591 levels that were localized to synapses with K562, versus 721-Cw4 cells (Figure 3A, p=0.0074). Hence, SHP-1 S591 phosphorylation is a regulated and directed event and may allow NK cells to locally regulate SHP-1 phosphorylation to enable the maintenance of multiple local activation states in a single cell.
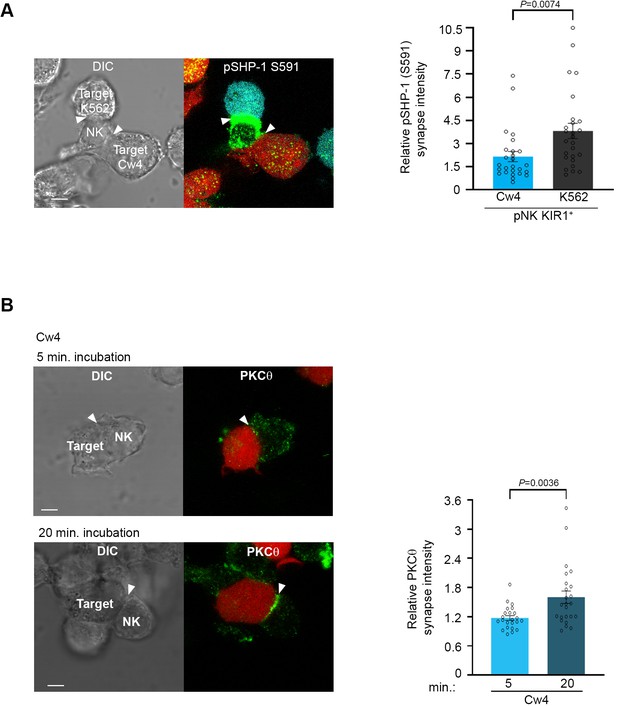
Phosphorylation of SHP-1 S591 occurs at the activating NKIS during simultaneous activating and inhibitory synapse formation, in parallel to PKC-θ accumulation.
(A) pNK-2DL1 cells were incubated over slides pre-seeded with 721-Cw4 and K562 cells expressing mCherry or CFP, respectively. The cells were incubated for 5 min at 37°C to enable conjugate formation and were fixed. pSHP-1 S591 was labeled with primary rabbit anti-pSHP-1 S591 antibody, and secondary anti-rabbit 488 antibody. Synapse intensity was quantified in NK cells relative to each cell in multiple NK cell synapses with two different targets (p=0.007, quantification on the right of triple-cell conjugates collected, n = 26). (B) YTS-2DL1 cells were incubated over slides pre-seeded with 721-Cw4 target cells expressing mCherry. The cells were incubated for 5 or 20 min at 37°C to enable conjugate formation and were fixed. PKC-θ was subsequently labeled with primary goat anti-PKC-θ antibody and secondary anti-goat 488 antibody. Right: graph summarizing PKC-θ accumulation at the NKIS at two time points following activation. Analysis was conducted comparing PKC-θ intensity at the NKIS relative to the rest of the NK cell. For Cw4, 5 and 20 min activation, n = 24 cell conjugates were analyzed from three independent experiments. Data are shown as mean ± SEM. One-sample t-tests (A, B) were used to calculate p-values.
-
Figure 3—source data 1
Numerical data for all graphical presentations in Figure 3.
- https://cdn.elifesciences.org/articles/73282/elife-73282-fig3-data1-v1.xlsx
We next aimed to identify the serine kinase implicated in SHP-1 S591 phosphorylation. PKC-θ is expressed predominantly in hematopoietic and muscle cells (Osada et al., 1992; Baier et al., 1993), and was shown to play multiple roles in T cell activation (Hayashi and Altman, 2007). In human NK cells, the role and molecular pathways of PKC-θ are incompletely defined. PKC-θ was suggested to participate in murine NK cell activity (Tassi et al., 2008; Aguiló et al., 2009; Anel et al., 2012; Merino et al., 2012); however, the mechanism by which it exerts this function remains unclear. It was shown that TNF-α and IFN-γ secretion is defective in PKC-θ−/− mice (Page et al., 2008), and this can contribute to defective recruitment of effector cells to the site of tumor development. In a different study (Tassi et al., 2008), no effect of PKC-θ deficiency was observed on IFN-γ secretion induced by IL-12, IL-18, or a combination of both cytokines. Therefore, it is not clear how PKC-θ is involved in the regulation of NK cell signaling cascades, and if its activity impacts human NK cell function.
Early reports by Vyas et al. showed that PKC-θ localizes to the cytolytic NK synapse during early activation, that is, following 5 and 10 min of activating target cell conjugation, and relatively low PKC-θ polarization is observed following 10 min of non-cytolytic NK: target cell conjugates (Vyas et al., 2002a). This PKC-θ localization was similar to the localization of SHP-1 at the NKIS. Thus, we next studied PKC-θ dynamics in NK cell inhibitory synapses and how these compare to PKC-θ localization during NK cell activation. Moreover, since PKC-θ plays a role in activation of different hematopoietic cells (Baier et al., 1993; Hayashi and Altman, 2007) and induces SHP-1 S591 phosphorylation during T cell activation (Liu et al., 2007), we tested its possible interplay with SHP-1 in NK cells. YTS-2DL1 NK cells were incubated with mCherry-labeled 721-Cw4 target cells for either 5 (early) or 20 (late) min (Figure 3B). PKC-θ localization appeared dispersed at the early inhibitory NKIS and accumulated at the synapse after 20 min (p=0.0036). These dynamics were similar to SHP-1 S591 phosphorylation patterns. Furthermore, we observed PKC-θ accumulation at the activating NKIS at the initial time point, which did not significantly decrease after 20 min (Figure 3—figure supplement 1, p=0.5068). This suggested that PKC-θ may play a role in regulating late inhibitory and early activating signaling pathways via SHP-1 regulation.
These data suggest that PKC-θ plays a role in human NK cell activation, and possibly in late (20 min) NK cell inhibition, potentially through SHP-1 regulation at the late inhibitory NKIS, and throughout the lifetime of the activating NKIS (5–20 min).
SHP-1 phosphorylation on S591 is facilitated through PKC-θ
In order to determine whether SHP-1 S591 phosphorylation is mediated through PKC-θ in NK cells, a specific small interfering RNA (siRNA) gene silencing approach was utilized in YTS-2DL1 and pNK-2DL1 cells. Cells were gene-silenced for PKC-θ and incubated with 721.221 target cells (Figure 4). Significant silencing efficiency was obtained in all experiments (p=0.001 and p=0.0135 for YTS and pNK-2DL1 cells, respectively) relative to cells transfected with nonspecific (NS) siRNA control (Figure 4A and C).
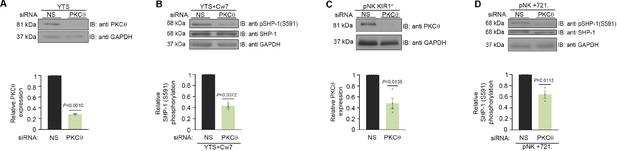
SHP-1 phosphorylation is mediated through PKC-θ.
(A) Silencing efficiency of PKC-θ. YTS-2DL1 cells treated with either nonspecific (control) (NS) or PKC-θ siRNA, lysed, separated on SDS-PAGE, and immunoblotted with anti- PKC-θ antibody. PKC-θ levels were measured by densitometric analysis, relative to the GAPDH loading control using ImageJ. Samples were normalized to the sample treated with NS siRNA (p=0.001, quantification on the bottom showing average of three independent experiments). (B) YTS-2DL1 treated with either NS or PKC-θ siRNA were incubated with 721- HLA target cells at 37°C for 5 min, and cells were subsequently lysed. Lysates were separated on SDS-PAGE, transferred to a nitrocellulose membrane, and immunoblotted with anti-pSHP-1 S591 antibody. SHP-1 S591 phosphorylation levels were measured by densitometric analysis, relative to the GAPDH loading control using ImageJ. Samples were normalized to the YTS-2DL1 sample treated with NS siRNA and incubated with 721-Cw7 targets (p=0.0072, quantification on the bottom of independent experiments, n = 3). (C) Silencing efficiency of PKC-θ. pNK-2DL1 cells were transfected with 250 pmol of PKC-θ siRNA. After 48 hr, prior to incubation with target cells, pNK-2DL1 were counted and lysed. Lysates were separated on SDS-PAGE and immunoblotted with anti-PKC-θ antibody. PKC-θ expression levels were measured by densitometric analysis using ImageJ and expressed relative to the GAPDH loading control. Samples were normalized according to the pNK-NS siRNA sample. Bar graph on the bottom shows the average of three independent experiments. (D) pNK-2DL1 cells were incubated with 721-HLA-negative cells at 37°C for 5 min, and cells were subsequently lysed. Lysates were separated on SDS-PAGE and transferred to a nitrocellulose membrane that was immunoblotted with anti-pSHP-1 S591 antibody. SHP-1 S591 phosphorylation levels were measured by densitometric analysis, relative to the GAPDH loading control using ImageJ. Samples were normalized to the pNK-2DL1 sample treated with NS siRNA and incubated with 721 targets (p=0.0113, quantification on the bottom showing the average of three independent experiments). Data are shown as mean ± SEM. One-sample t-tests (A–D) were used to calculate p-values.
-
Figure 4—source data 1
Representative blots.
- https://cdn.elifesciences.org/articles/73282/elife-73282-fig4-data1-v1.pdf
-
Figure 4—source data 2
Numerical data for all the graphical presentations in Figure 4.
- https://cdn.elifesciences.org/articles/73282/elife-73282-fig4-data2-v1.xlsx
Next, SHP-1 S591 phosphorylation levels were examined in YTS-2DL1 and pNK-2DL1 cells that were treated with NS siRNA vs. PKC-θ siRNA and incubated with 721-Cw7 or 721-HLA-negative target cells in order to elucidate the role of PKC-θ on SHP-1 S591 phosphorylation during stimulation. Though S591 phosphorylation was not completely eliminated, a reduction in SHP-1 S591 phosphorylation levels could be seen in YTS-2DL1 or pNK-2DL1 treated with PKC-θ siRNA as opposed to NS siRNA (by 2.5-fold ± 0.03, p=0.0072; by 1.7-fold ± 0.07, p=0.0113, respectively) (Figure 4B and D). Together, these data demonstrate PKC-θ involvement in SHP-1 S591 phosphorylation in NK cells.
PKC-θ regulates SHP-1 conformation status and its enzymatic activity at the NKIS
To determine whether SHP-1 conformation is affected by PKC-θ, YTS-2DL1 cells were co-transfected with YFP-SHP-1-CFP along with NS or PKC-θ -specific siRNA and incubated with targets for 5 min. Synaptic FRET efficiency was significantly reduced in YTS-2DL1 YFP-SHP-1-CFP cells pretreated with PKC-θ siRNA versus NS siRNA (18.5% ± 2.04% vs. 9.8% ± 1.7%, p=0.0025), suggesting that the SHP-1 in the silenced cells acquires the open conformation (Figure 5A and B). Interestingly, PKC-θ gene silencing reduced the FRET efficiency of YFP-SHP-1-CFP in the activating NKIS to similar FRET levels observed in YTS-2DL1 YFP-SHP-1-CFP cells pretreated with NS siRNA following inhibitory interactions (5.76% ± 1.6% vs. 9.8% ± 1.7%, p=0.2663) (Figure 5A and B). Hence, although the SHP-1 conformation in activating synapses is closed and inactive, PKC-θ gene silencing increases SHP-1 open and active conformation at the activating NKIS, as detected by reduced FRET efficiency between the N′ and C′ termini of the SHP-1 sensor. This may suggest a role for PKC-θ in reducing SHP-1 activity at the activating NKIS, where SHP-1 localizes yet is inactive, and possibly during the termination of the inhibitory synapse to enable subsequent NK cell activity.
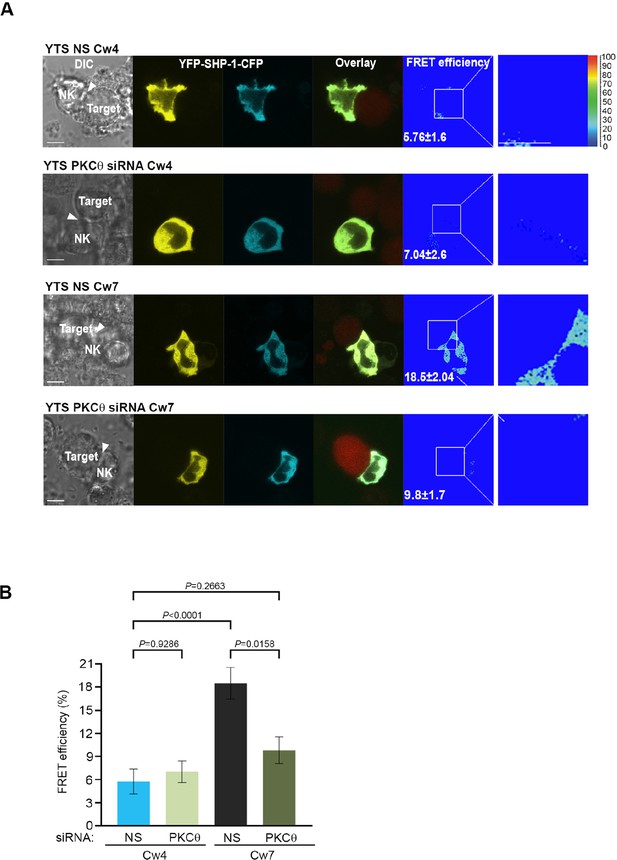
SHP-1 conformation is regulated by PKC-θ at the NKIS.
(A) YTS-2DL1 YFP-SHP-1-CFP cells were treated with either NS or PKC-θ siRNA and incubated over slides pre-seeded with 721-Cw4 (top panels) or Cw7 (bottom panels) target cells expressing mCherry. The cells were incubated for 5 min at 37°C to enable conjugate formation and were fixed. FRET analysis was performed, as indicated. (B) Graph summarizing FRET efficiency during 5 min activation with 721-Cw4 or Cw7 target cells. For Cw4 NS and PKC-θ siRNA, n = 52 and 63 cell conjugates were analyzed, respectively, and for Cw7 NS and PKC-θ siRNA n = 67 and 61 cell conjugates were analyzed from three independent experiments, respectively. Data are shown as mean ± SEM. Two-way ANOVA with Tukey test (B) was used to calculate p-values.
-
Figure 5—source data 1
Numerical data for all the graphical presentations in Figure 5.
- https://cdn.elifesciences.org/articles/73282/elife-73282-fig5-data1-v1.xlsx
In order to examine whether the altered SHP-1 conformation induced under PKC-θ gene silencing affects SHP-1 catalytic activity, SHP-1 activity was assessed through PTP assay. Direct catalytic activity of SHP-1 on the pNPP substrate was shown to be higher in cells treated with PKC-θ siRNA rather than in cells treated with NS siRNA (81% ± 3.3% vs. 65% ± 3% activity, p=0.0246) (Figure 5—figure supplement 1). Collectively, these results indicate that SHP-1 phosphorylation by PKC-θ can shift the SHP-1 conformational state from open (active) to closed (inactive), and thus reduce SHP-1 enzymatic activity.
SHP-1 activity is modified under PKC-θ-mediated regulation
To further characterize the ability of PKC-θ to regulate SHP-1 catalytic activity, the phosphorylation profile of key signaling proteins that serve as SHP-1 substrates was compared following NK cell activation or inhibition under PKC-θ gene silencing. SHP-1 was shown to dephosphorylate VAV1 as a mechanism of terminating NK cell activation (Stebbins et al., 2003). Furthermore, our recent studies identified novel SHP-1 substrates including PLCγ1/2 and LAT (Matalon et al., 2016).
We therefore examined how PKC-θ gene silencing affects SHP-1 catalytic activity on its substrates in YTS and pNK-2DL1 cells. In order to assess the phosphorylation status of these proteins, cells were treated with either PKC-θ siRNA or NS siRNA and incubated with inhibitory Cw4-721 or activating Cw7-721 or 721-HLA-negative target cells. IB (immunoblotted) of pVAV1 (Y160) revealed an approximately twofold reduction in phosphorylation levels in activated YTS-2DL1 samples treated with PKC-θ siRNA in contrast to NS siRNA (p=0.0001) (Figure 6A). Similarly, pNK-2DL1 cells demonstrated a similar reduction in pVAV1 (Y160) phosphorylation levels subsequent to PKC-θ gene silencing (p=0.0192) (Figure 6A). Furthermore, phosphorylation of VAV1 in activating interactions following PKC-θ gene silencing was similar to its phosphorylation during inhibitory interactions (YTS and pNK-2DL1 cells treated with NS siRNA). In addition to VAV1, pNK-2DL1 cells showed a 1.8-fold ± 0.2 reduction in pPLCγ1 levels (p=0.0032) when treated with PKC-θ siRNA (Figure 6B). A similar trend can be seen in YTS-2DL1 cells in which immunoprecipitation (IP) of PLCγ1 and IB of pPLCγ1 (Y783) revealed a reduction of 1.4-fold ± 0.04 in pPLCγ1 levels following PKC-θ gene silencing under activating interactions (p=0.0074) (Figure 6E). Together, these data suggest that PKC-θ-mediated regulation of SHP-1 catalytic activity may promote SHP-1 inactivation, and thus enhance NK cell reactivity.
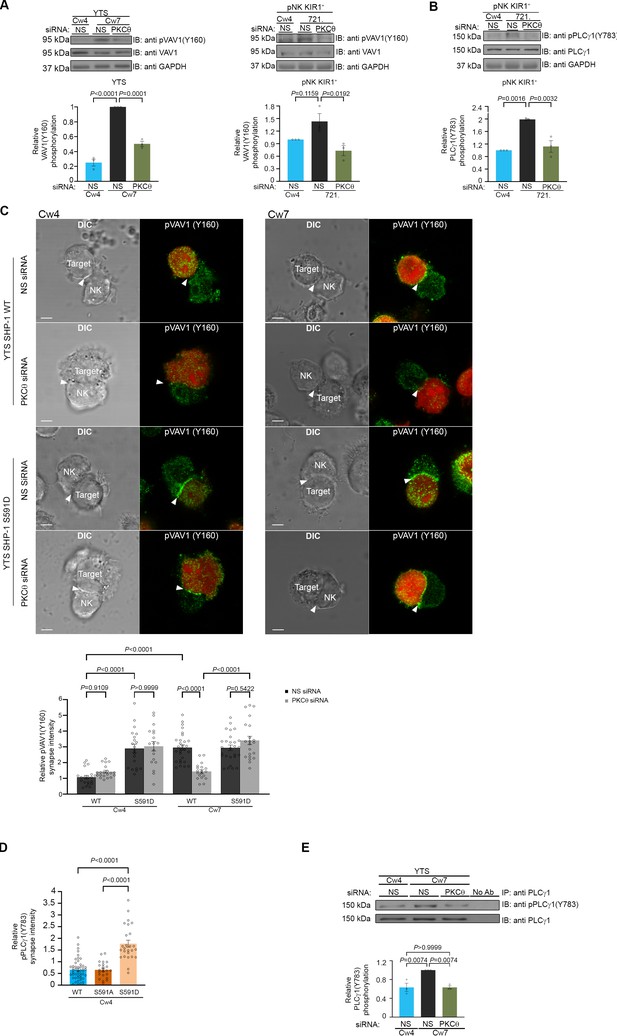
SHP-1 substrate phosphorylation is reduced following PKC-θ silencing.
(A) YTS-2DL1 or pNK-2DL1 cells were transfected with 500 pmol of PKC-θ siRNA for 48 hr. Cells were incubated with target cells for 5 min at 37°C and then lysed. Lysates were separated on SDS-PAGE and immunoblotted with anti-pVAV-1 (Y160) antibody. Phosphorylation levels were measured by densitometric analysis, relative to the GAPDH loading control using ImageJ. Samples were normalized according to the YTS-2DL1 or pNK-2DL1 NS siRNA Cw4 sample. Quantification of independent experiments is shown on the bottom; n = 3 for YTS and n = 4 for pNK experiments. (B) pNK-2DL1 cells were transfected with either NS or PKC-θ siRNA 48 hr prior to each experiment. Cells were incubated with either 721-Cw4 or 721-HLA-negative target cells for 5 min at 37°C, and subsequently lysed. Lysates were separated on SDS-PAGE and immunoblotted with anti-pPLCγ-1 (Y783) antibody. Phosphorylation levels were measured by densitometric analysis, relative to the GAPDH loading control using ImageJ. Samples were normalized to the pNK-2DL1 NS siRNA Cw4 sample. Quantification of independent experiments is shown on the bottom; n = 3. The blot in (A) was stripped and reblotted against pPLCγ1(Y783) in (B). (C) YTS-2DL1 WT or YTS-2DL1 SHP-1 S591D cells were transfected with either NS or PKC-θ siRNA and incubated over slides pre-seeded with 721-Cw4 or Cw7 target cells expressing mCherry. The cells were incubated for 5 min at 37°C to enable conjugate formation and fixed. pVAV-1 (Y160) was subsequently labeled with primary rabbit anti-pVAV-1 (Y160) antibody and secondary anti-rabbit 488 antibody. Quantification is shown on the bottom; for YTS-2DL1 WT NS or PKC-θ siRNA vs. Cw4 and Cw7, n = 21 and 28 cell conjugates were analyzed, respectively. For YTS-2DL1 SHP-1 S591D NS or PKC-θ and Cw7, n = 21 and 28 cell conjugates were analyzed from three independent experiments, respectively. (D) YTS-2DL1 SHP-1 KO cells were transfected with either WT YFP-SHP-1, YFP-SHP-1 S591A, or YFP-SHP-1 S591D phosphorylation mutants, and incubated on slides with mCherry-expressing 721-Cw4 target cells at 37°C. After 5 min incubation, the cells were fixed and stained with anti-pPLCγ1(Y783). NK cells were distinguished from targets based on mCherry expression by the target cells. Graph summarizes the relative synapse staining intensities. For WT YFP-SHP-1, YFP-SHP-1 S591A, and YFP-SHP-1 S591D, n = 48, 48, and 25 cell conjugates from three independent experiments analyzed, respectively. (E) YTS-2DL1 cells were transfected with either NS or PKC-θ siRNA 48 hr prior to the experiment. Cells were incubated with either 721-Cw4 or Cw7 target cells for 5 min at 37°C and subsequently lysed. Lysates were immunoprecipitated on beads containing PLCγ-1 antibody and immunoblotted for pPLCγ-1 (Y783). Densitometric analysis was normalized to PLCγ-1 loading controls, and relative to the YTS-2DL1 NS siRNA: Cw7 pPLCγ-1(Y783) sample. Bar graph on the bottom shows the average of three independent experiments. Data are shown as mean ± SEM. One-way ANOVA with Tukey test (A, B, D, E) or two-way ANOVA with Tukey test (C) was used to calculate p-values.
-
Figure 6—source data 1
Representative blots.
- https://cdn.elifesciences.org/articles/73282/elife-73282-fig6-data1-v1.pdf
-
Figure 6—source data 2
Numerical data for all the graphical presentations in Figure 6.
- https://cdn.elifesciences.org/articles/73282/elife-73282-fig6-data2-v1.xlsx
In order to demonstrate that PKC-θ regulation of SHP-1 via S591 influences SHP-1 substrate phosphorylation and activation, and not PKC-θ silencing per se, a mutant YTS-2DL1 line expressing an SHP-1 mutant that mimics the constitutively phosphorylated state, SHP-1 S591D (referred to as YTS-2DL1 SHP-1 S591D), was created utilizing CRISPR/Cas9, as previously reported (Ben-Shmuel et al., 2021). In cells expressing this mutant, PKC-θ silencing would not be expected to impact SHP-1 activity (Egelhoff et al., 1993; Huang and Erikson, 1994; Léger et al., 1997). Hence, we expected that the S591D mutant would demonstrate high accumulation of pVAV1 in the synapse, irrespective of PKC-θ silencing. WT YTS-2DL1 or YTS-2DL1 SHP-1 S591D NK cells were treated with either NS or PKC-θ siRNA, and incubated with mCherry-expressing target cells to assess pVAV1 Y160 synaptic accumulation. As expected, pVAV1 Y160 accumulation was observed during activating but not inhibitory NK cell interactions (p<0.0001), and in accordance with our results, PKC-θ siRNA-treated NK cells had a 2 ± 0.2-fold reduction in pVAV1 recruitment to the activating NKIS (p<0.0001), indicating increased SHP-1 activity in the absence of PKC-θ under activating interactions (Figure 6C). No significant decrease in pVAV1 recruitment was observed, however, in YTS-2DL1 SHP-1 S591D cells treated with either NS or PKC-θ siRNA under either activating or inhibitory interactions (p=0.9109 and p=0.9999, respectively). Furthermore, YTS-2DL1 SHP-1 KO cells were reconstituted with either YFP-SHP-1 WT or with YFP-SHP-1 mutant constructs, including the constitutively phosphorylated SHP-1 mimetic (YFP-SHP-1 S591D, constitutively inactive) or an SHP-1 phospho-abolishing mutant (YFP-SHP-1 S591A, constitutively active). YTS-2DL1 SHP-1 KO cells transfected with the YFP-SHP-1 constructs were incubated with Cw4-721 targets expressing mCherry and stained for pPLCγ1 (Y783) (Figure 6D). We previously showed that pPLCγ1 (Y783) accumulation at the NKIS is favored during activating rather than inhibitory NK cell interactions (Matalon et al., 2016). Interestingly, cells expressing SHP-1 S591D showed high levels of pPLCγ1 (Y783) accumulation at the NKIS, even though the NKIS was inhibitory. These levels were approximately three-fold higher compared to YTS-2DL1 cells expressing SHP-1 WT or SHP-1 S591A (p<0.0001). Collectively, these results demonstrate that PKC-θ negatively regulates SHP-1 activity by phosphorylating S591, as demonstrated through higher VAV1 and PLCγ1 tyrosine phosphorylation, and accumulation at the NK synapse.
PKC-θ-mediated regulation of SHP-1 augments NK cell activation
SHP-1 tunes NK cell activation, and, as we demonstrated here, PKC-θ-mediated phosphorylation of SHP-1 impacts its enzymatic activity. We therefore next determined the effect of PKC-θ regulation on NK cell effector function and activation. PKC-θ silencing in NK cells resulted in a decrease in the phosphorylation profile of VAV1 and PLCγ1 in a SHP-1-dependent manner (Figure 6). These data suggest that the PKC-θ:SHP-1 axis may affect NK cell activation and cytotoxicity in a manner that is highly dependent on calcium flux and actin reorganization (Orange et al., 2002; Stebbins et al., 2003; Bryceson et al., 2006; Caraux et al., 2006; Upshaw et al., 2006; Andzelm et al., 2007; Kim et al., 2010; Dong et al., 2012; Mizesko et al., 2013; Carisey et al., 2018). To this end, PKC-θ was gene-silenced in YTS-2DL1 cells, and intracellular calcium flux was measured in NK cells incubated with activating 721-Cw7 target cells. As expected, calcium flux levels were higher in activated vs. inhibited NK cells treated with NS siRNA (black vs. blue curve, Figure 7A). However, PKC-θ siRNA reversed this trend, reducing calcium flux levels during NK cell activation near the levels obtained during NK cell inhibition (green vs. blue curve, Figure 7A). Similar results were obtained in pNK-2DL1 cells transfected with NS or PKC-θ siRNA and incubated with activating 721-HLA-negative or 721-Cw4-expressing cells (Figure 7—figure supplement 1). These results are consistent with the reduced phosphorylation levels of the calcium regulator, PLCγ1 (Figure 6B, D and E ).
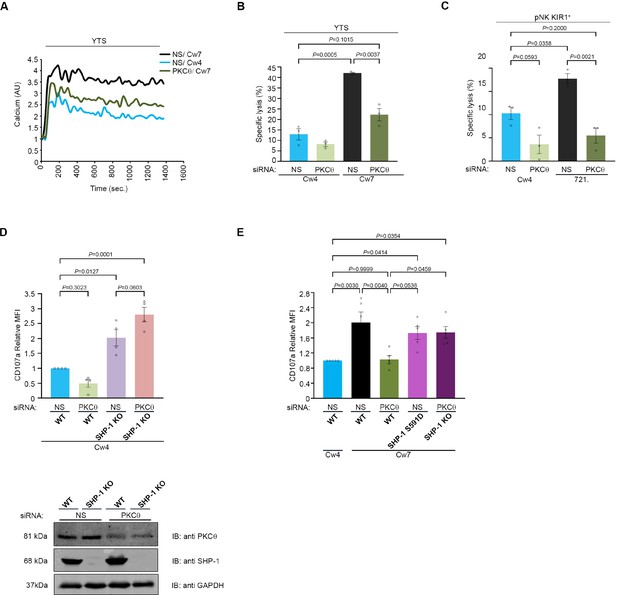
NK cell activation threshold is increased following gene silencing of PKC-θ.
YTS-2DL1 cells were transfected with specific PKC-θ siRNA or with NS siRNA and assessed for effector activity after 48 hr. (A) YTS-2DL1 cells were loaded with calcium-sensitive Fluo-3-AM and analyzed for basal intracellular calcium levels for 1 min. The NK cells were then mixed with 721-Cw4 or Cw7 target cells and incubated at 37°C and analyzed by spectrofluorometry. (B) YTS-2DL1 cells were incubated with [35S]Met-labeled 721-Cw4 or Cw7 target cells at a ratio of 10:1 for 5 hr at 37°C. The specific lysis of target cells was measured. The graph summarizes three independent experiments. (C) pNK-2DL1 cells were incubated with [35S]Met-labeled 721-Cw4 or HLA-negative target cells at a ratio of 10:1 for 5 hr at 37°C. The specific target cell lysis was measured. The graph shows the average of three independent experiments. (D) WT YTS-2DL1 or YTS-2DL1 SHP-1 KO cells were treated with either NS or PKC-θ-specific siRNA 24 hr before incubation with mCherry-expressing 721-Cw4 target cells for 2 hr at 37°C, and analyzed for degranulation via FACS relative to the WT NS-treated cells incubated with 721-Cw4 targets, as described in Materials and methods. A representative blot is shown (bottom) demonstrating PKC-θ and SHP-1 expression. (E) WT YTS-2DL1, YTS-2DL1 SHP-1 KO, and YTS-2DL1 SHP-1 S591D NK cells were treated with PKC-θ or NS siRNA and were incubated with mCherry-expressing 721-Cw4 or 721-Cw7 target cells for 2 hr at 37°C and analyzed for degranulation via FACS. Degranulation was assessed relative to the WT NS-treated cells incubated with 721-Cw4 targets, as described in Materials and methods. For knockdown, cells were treated with either NS or PKC-θ-specific siRNA 24 hr before incubation with mCherry-expressing 721-Cw4 or 721-Cw7 target cells; the graph shows the average of four independent experiments. Data are shown as mean ± SEM. Two-way ANOVA with Tukey test (B, C) or one-way ANOVA with Tukey test (D, E) was used to calculate p-values.
-
Figure 7—source data 1
Numerical data for all the graphical presentations in Figure 7.
- https://cdn.elifesciences.org/articles/73282/elife-73282-fig7-data1-v1.xlsx
-
Figure 7—source data 2
Representative blots.
- https://cdn.elifesciences.org/articles/73282/elife-73282-fig7-data2-v1.pdf
To assess how the PKC-θ:SHP-1 regulation controls NK cell cytotoxic potential, YTS-2DL1 cells were gene-silenced for PKC-θ and incubated with 721-Cw4 or Cw7 target cells, and target cell lysis was measured through Met release assay [35S], a direct measurement of NK cell killing of cancerous cells (Figure 7B). Target cell death was significantly reduced in YTS-2DL1 samples that were gene-silenced for PKC-θ under activating interactions (22% ± 3% vs. 42% ± 0.4%, p=0.0037). In fact, activating target cells (Cw7) incubated with PKC-θ-silenced NK cells demonstrated lysis levels similar to those of inhibitory targets (Cw4) incubated with YTS-2DL1 cells treated with NS siRNA (22% ± 3% vs. 13% ± 2.7%, p=0.1015). These data were verified in human pNK-2DL1 cells treated with NS or PKC-θ siRNA (5.5% ± 1.6% vs. 18% ± 1.06%, p=0.0021, and 5.5% ± 1.6% vs. 10% ± 1.32%, p=0.2) (Figure 7C, Figure 7—figure supplement 1). To verify that absence of PKC-θ influences NK cell effector functions through SHP-1 activity, and that the effects of PKC-θ silencing are abrogated in the absence of SHP-1, SHP-1 KO NK cells were additionally gene-silenced for PKC-θ (Figure 7D). Subsequently, WT and SHP-1 KO NK cells treated with either NS or PKC-θ siRNA were subjected to CD107a degranulation assay. As can be seen in Figure 7D, NK cells that were deficient in SHP-1 or both SHP-1 and PKC-θ displayed higher degranulation than WT cells (p=0.0127 and p=0.0001) and were not significantly affected by the presence or absence of PKC-θ (p=0.0603). Furthermore, as shown in Figure 7E, PKC-θ knockdown also inhibits the NK cell-mediated antitumor response against 721-Cw7 cells as demonstrated by reduced NK cell degranulation compared to YTS-2DL1 cells treated with NS siRNA (p=0.0040). Additionally, YTS-2DL1 SHP-1 S591D and YTS-2DL1 SHP-1 KO cells treated with NS or PKC-θ siRNA demonstrated restored degranulation (p=0.04) against activating target cells, suggesting that antitumor responses were rescued by the absence of SHP-1 or the SHP-1 mutation at the PKC-θ phosphorylation site. Collectively, these data demonstrate that PKC-θ negatively regulates SHP-1 conformational state and activity, thereby providing a mechanism for maintaining NK cell activation and cytotoxic potential.
SHP-1-deficient NK cells are unaffected by PKC-θ silencing and promote superior antitumor clearance relative to WT cells
We recently demonstrated that abrogation of SHP-1 activity in engineered NK cells can have enhanced benefits for NK-based immunotherapeutic approaches (Ben-Shmuel et al., 2021).Furthermore, it was recently shown that lower abundance of SHP-1 in NK cells enhances their tumoricidal capacity (Wu et al., 2021). To verify that NK cells silenced for PKC-θ have lower antitumor cytotoxicity due to enhanced SHP-1 activity, and not due to the absence of PKC-θ per se, non-obese diabetic (NOD) Rag1nullIL2Rγnull (NRG) and NOD-SCID IL2Rγnull (NSG) mice were subcutaneously engrafted with 721-Cw4 or 721-Cw7 tumor cells, and injected with either 5 × 106 WT YTS-2DL1, WT YTS-2DL1 gene-silenced for PKC-θ, YTS-2DL1 SHP-1KO cells (Matalon et al., 2018), YTS-2DL1 SHP-1KO cells that were gene-silenced for PKC-θ or YTS-2DL1 SHP-1 S591D mutant cells (Ben-Shmuel et al., 2021), every 3 days for a total of six treatments (Figure 8A). Tumor volumes were monitored daily to examine the effect on tumor size and average growth rate (Figure 8B–E). If PKC-θ affects NK cell cytotoxicity through a dominant alternative pathway to SHP-1, then we would expect the observed phenotype of SHP-1 silenced/mutated NK cells (highly increased killing and arrest of tumor growth) to be abrogated, at least partially, upon PKC-θ silencing. Indeed, mice injected with YTS-2DL1 SHP-1-deficient/catalytically inactive cells that were treated with PKC-θ siRNA demonstrated slower tumor growth rates and smaller tumor volumes compared to mice injected with WT NK cells (Figure 8). In order to focus on the NK response toward targets cells that induce activating signals and demonstrate the involvement of PKC-θ-mediated SHP-1 phosphorylation in NK cell cytotoxicity, mice were subcutaneously engrafted with 721- Cw7 cells and injected as described above. As expected, PKC-θ-silenced NK cells exhibited decreased antitumor activity toward activating target cells (721-Cw7), while mice engrafted with YTS-2DL1 SHP-/- gene-silenced to PKC-θ exhibited a stronger antitumor response with lower tumor growth rates, suggesting that loss of SHP-1 activity restored the NK antitumor responses (p<0.0001, Figure 8D and E, Figure 8—figure supplement 1). These results suggest that regulation of SHP-1 through PKC-θ impacts NK cell cytotoxicity and affects NK cell activity for in vivo tumor clearance.
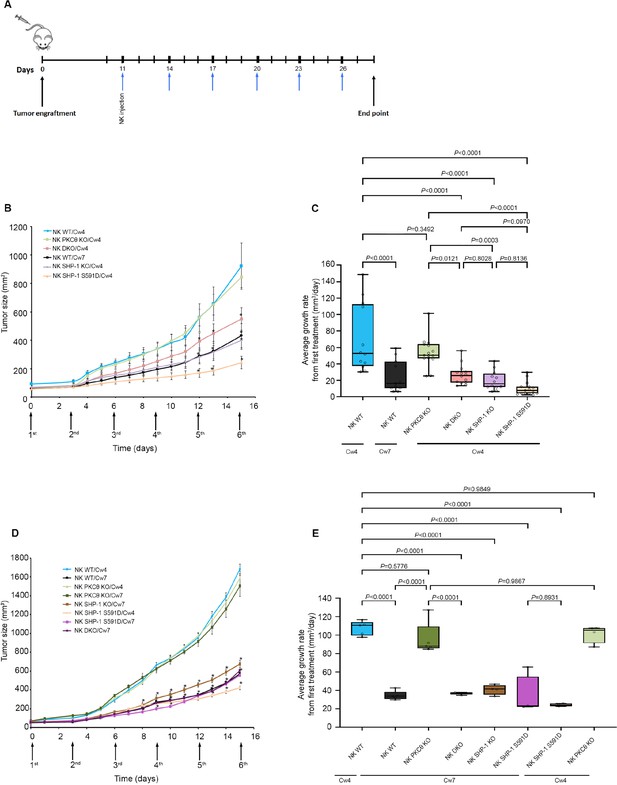
SHP-1-deficient NK cells exhibit enhanced antitumor activity in vivo, irrespective of PKC-θ expression.
(A) Schematic representation of the experimental timeline. (B) Tumor volumes measured daily in NOD-Rag1nullIL2rgnull (RAG) mice. NOD-Rag1nullIL2rgnull (RAG) mice were subcutaneously injected with 3 × 106 721-Cw4 or Cw7-expressing tumor cells. Mice were administered by intratumor injections every 3 days with either 5 * 106 irradiated WT YTS-2DL1, WT YTS-2DL1 that were treated with PKC-θ siRNA (NK PKC-θ KO), YTS-2DL1 SHP-1 KO cells (NK SHP-1 KO), YTS-2DL1 SHP-1 KO cells that were treated with PKC-θ siRNA (NK DKO), or YTS-2DL1 SHP-1 S591D mutant cells. (C) Average growth rates of tumors from the first treatment to the end point. Tumor volumes and tumor growth rates were calculated as described in Materials and methods. Data are shown as mean ± SEM. Two-way ANOVA with Tukey test (B, C) was used to calculate p-values. (D) Mice were subcutaneously injected with 3 × 106 721-Cw4 or Cw7-expressing tumor cells. Mice were treated by intratumor injections every 3 days with either 5 * 106 irradiated WT YTS-2DL1, WT YTS-2DL1 PKC-θ KO, YTS-2DL1 SHP-1 KO, YTS-2DL1 DKO, or YTS-2DL1 SHP-1 S591D mutant cells (n = 5). Tumor volumes were measured daily. (E) Average growth rates of tumors from the first treatment to the end point. Tumor volumes and tumor growth rates were calculated as described in Materials andethods. Data are shown as mean ± SEM. Two-way ANOVA with Tukey test (D, E) was used to calculate values.
-
Figure 8—source data 1
Numerical data for all the graphical presentations in Figure 8.
- https://cdn.elifesciences.org/articles/73282/elife-73282-fig8-data1-v1.xlsx
Discussion
SHP-1 plays a central role in the regulation of the NK cell activation threshold (Stebbins et al., 2003; Lanier, 2005; Long, 2008; Matalon et al., 2016) and is critical for the NK cell education process (Viant et al., 2014). Importantly, activation of SHP-1 and its homolog SHP-2 (an additional critical inhibitory NK cell regulatory enzyme) (Purdy and Campbell, 2009) are the major mechanisms operating downstream to immune checkpoint receptors, such as PD-1, CTLA-4, BTLA, and LAG-3 (Lee et al., 1998; Watanabe et al., 2003; Sheppard et al., 2004; Huang et al., 2015). It is therefore of great interest to understand how this important regulator is kept in check during NK cell activation and inhibition as this regulatory mechanism may have critical consequences for additional immune cells, immune checkpoint pathways, and immunotherapies.
NK cell activity is regulated by a balance between stimulatory or inhibitory signals, yet how these signals are integrated and how intracellular NK cell activation is maintained and controlled through these multiple receptors remain incompletely understood. Moreover, the presence of SHP-1 at the activating NKIS and rapid dispersal from the inhibitory NKIS (Vyas et al., 2002a) suggest as yet unknown roles and regulatory mechanisms. Here, we elucidate some of these unresolved mechanisms by showing that the NK cell activation threshold is maintained through downregulation of SHP-1 phosphatase activity regulated by PKC-θ.
Studies published on outcomes of SHP-1 C′ terminal serine phosphorylation in non-NK cell systems demonstrated contradicting results (Li et al., 1995; Brumell et al., 1997; Jones et al., 2004; Poole and Jones, 2005; Liu et al., 2007). Here, we demonstrate that phosphorylation of SHP-1 by PKC-θ during NK cell activation on serine 591 maintains heightened NK cell effector function by retaining the SHP-1 closed and inactive conformation. This mechanism preserves SHP-1 substrate phosphorylation and consequently sustains calcium flux and cytotoxicity. Thus, the PKC-θ:SHP-1 axis may play an important role in NK immune surveillance and tumor clearance, a topic that has recently gained much attention in the immunotherapy field, especially in the context of adoptive cell transfer and genetically engineered NK cells (Dotta et al., 2007; Morandi et al., 2008; Ahern and Brennan, 2011; Jochems et al., 2016; Siegler et al., 2017).
Our findings may answer an important question regarding how SHP-1 activity is downmodulated after dissociation of the inhibitory NKIS, and how its signaling is downregulated during cytolytic NK responses. Phosphorylation of SHP-1 during termination of the inhibitory NKIS may retain the activity of NK cells to subsequent targets, whereas its immediate phosphorylation at the activating NKIS maintains the NK cell lytic capacity. This is consistent with the SHP-1 conformation patterns shown here (Figure 2) within the activating and inhibitory NKIS.
Interestingly, mathematical modeling analysis in NK cells suggests that weak ligands for activating receptors (bearing partially phosphorylated ITAMs) can recruit SHP-1 and thereby increase the NK activation threshold, while under certain conditions (such as low concentration of SHP-1 and weak activating ligands) inhibitory receptors can aid in NK cell activation (Das, 2010). Thus, it is possible that one mechanism facilitating target cell escape from NK cell lysis is selection for cells expressing weak activating ligands that induce SHP-1 recruitment. It is clear that the transition of SHP-1 between inhibiting and activating receptors must be tightly regulated to ensure proper NK cell responses.
PKC-θ is the only PKC isoform that was shown to accumulate at T cell and NKISs (Monks et al., 1997; Merino et al., 2012). In T cells, it is clear that PKC-θ plays an important role in T cell activation and survival by activating several downstream pathways, including NF-kB and AP-1 as major targets (Wang et al., 2015). In addition, Merino et al. previously showed that clustering of PKC-θ at the NKIS amplifies murine NK cell activation and effector functions (Merino et al., 2012), but the mechanism by which it exerts this function and specifically in human cells remained mostly unknown. Several studies suggest the involvement of PKC-θ in signal transduction, antitumoral activity, and NK cell degranulation (Anel et al., 2012). Here, we demonstrate that in human NK cells PKC-θ phosphorylates SHP-1 on S591 (Figure 4, Figure 3—figure supplement 1), suppresses SHP-1 activity, and thereby increases NK cell activity. PKC-θ appears to regulate SHP-1 activity during the late inhibitory synapse and through the full duration of activating NK:target cell interactions. Indeed, gene silencing of PKC-θ resulted in the closed SHP-1 conformation (Figure 5), implying that SHP-1 favors an open and active conformation in the absence of PKC-θ. This conformational change in SHP-1 also impacts its catalytic activity. These findings were strengthened through in vivo experiments, which demonstrated that absence of SHP-1 increased NK cell activity and decreased tumor progression, irrespective of PKC-θ expression, implying that PKC-θ indeed maintains NK cell activation through its regulation of SHP-1. PKC-θ also plays a role in NF-κB activation as shown by Sun et al., 2000. Thus, PKC-θ knockout could possibly affect NF-κB transcriptional activity, resulting in a reduced antitumor immune response. As shown in Figures 7E and 8D and E, YTS-2DL1 cells gene-silenced for PKC-θ show reduced degranulation, resulting in increased tumor growth rates, whereas YTS-2DL1 SHP-1 KO cells treated with PKC-θ siRNA (DKO) show increased degranulation and reduced tumor growth rate relative to YTS-2DL1 cells gene-silenced for PKC-θ, suggesting that SHP-1 knockout rescues the antitumor response. This result confirms the role of PKC-θ in regulating SHP-1 activity. It seems that a dynamic and coordinated balance between activating and inhibitory signaling cascades governs NK cell activation programs. Das et al. proposed that NK cell tolerance or activation is modulated by signal strength of the target cells (Das, 2010), which is in line with the suggestion that NK cell regulation occurs through a balance of activating and inhibitory signals transmitted by membrane receptors recognizing potential ligands on the target cell surface (Lanier, 2001). Here, we elucidate the potential circuitry between kinase and phosphatase activities playing a pivotal role in balancing activating and inhibitory NK signaling. Several studies show that deficiency or inhibition of PKC-θ could potentially decrease the severity of autoimmunity, allergy, and chronic inflammation. For example, in animal models of intestinal inflammatory disease (chronic colitis), PKC- θ KO mice showed decreased T cell proliferation and cytokines production (Chand et al., 2012; Curnock et al., 2014; Nicolle et al., 2021), suggesting that PKC-θ inhibitors could be useful as a therapeutic approach for inflammatory disorders (Chand et al., 2012; Curnock et al., 2014).
Though pSHP-1 levels were substantially reduced after PKC-θ gene silencing, basal levels were still evident. It is possible that other serine/threonine kinases or isoforms such as PKC-θ may also be involved in this process (Jones et al., 2004). Another possible mechanism may involve PKC-θ cross talk with the NK cytoskeleton. We recently demonstrated that SHP-1 conformation and activity is dependent on the association to the cytoskeletal machinery and cytoskeletal dynamics (Matalon et al., 2018). One possibility is that cross-talk between PKC-θ and SHP-1 mediates actin:SHP-1 binding or disassociation, thereby regulating SHP-1 catalytic activity and conformation. Furthermore, PKC-θ phosphorylates the Wiskott-Interacting Protein (WIP) in NK cells upon activation, and this facilitates recruitment of actin and myosin IIA to WIP and WASp for the formation of the multiprotein complex (Krzewski et al., 2006).
After SHP-1 mediates its function and prevents NK cell activity during an inhibitory interaction, its activity should be downregulated to enable subsequent NK cell interactions with activating susceptible targets. This can be seen by late phosphorylation (after 20 min) of SHP-1 S591 during inhibitory NKISs (Figure 1), and may serve as a mechanism of kinetic priming, ensuring that NK cell activity remains stable for future contacts (Choi and Mitchison, 2013).
Recently, it was shown that as NK cells are constantly situated in an environment that requires simultaneous killing of target cells and sparing of others, accurate and specific convergence of lytic granules aids in killing only relevant targets, while sparing healthy cells (Hsu et al., 2016). Moreover, a study by Netter et al. demonstrated how initiation and termination of the activating NKIS are highly regulated and enable serial NK cell killing by accelerating detachment from one target cell and simultaneous formation of new cytotoxic NKISs (Netter et al., 2017). Additional reports by Srpan et al. describe NK cell serial killing via shedding of surface receptors (Srpan et al., 2018). Thus, sequential and highly regulated signaling is needed for NK cell maneuverability in a complex immune environment. PKC-θ: SHP1 regulation may enable an NK cell to rapidly survey its surroundings and maintain its effector functions in a complex immune environment that requires simultaneous sparing of normal cells and killing of malignant targets. This mechanism would also ensure that NK cell effector functions are maintained in the presence of an activating NKIS. Cancer cells can express MHC-I and other inhibitory NK cell ligands; thus, recruitment and activation of PKC-θ at the activating NKIS may limit SHP-1 association to ITIMs that could prevent tumor cell killing. It would be interesting to assess the effect of S591 phosphorylation on SHP-1:KIR ITIM association as this phosphorylation may not only affect the NK cell activation threshold, but also NK cell education, as SHP-1 was shown to be vital for regulating NK cell responsiveness to tumors (Wu et al., 2021).
Due to the fact that NK cells are also implicated in autoimmune disorders such as multiple sclerosis (Morandi et al., 2008), rheumatoid arthritis (Ahern and Brennan, 2011), and type I diabetes (Dotta et al., 2007), deregulation of SHP-1 activity may be a factor affecting normal NK cell functions against healthy cells. SHP-1 was also shown to serve as an important regulator that impacts cell proliferation and survival (Chong and Maiese, 2007). Some studies examined its role in tumor transformation (Wu et al., 2003). It would be interesting to examine whether mutations or regulation of SHP-1 S591 or other known or as yet unidentified residues may impact its function. Understanding regulatory protein circuits in NK cells will be an important step for further elucidating complex pathways such as NK signaling cascades and NK cell education, and potentially open doors to innovative immunotherapies shaping NK cell behavior.
Materials and methods
Reagent type (species) or resource | Designation | Source or reference | Identifiers | Additional information |
---|---|---|---|---|
Antibody | Anti-human PLCγ1 (mouse monoclonal) | Upstate | 05-163 | IB: 1:500 (14 µL) |
Antibody | Anti-human VAV1 (D7) (mouse monoclonal) | Santa Cruz | SC-8039 | IB: 1:500 (14 µL) |
Antibody | Anti-human SHP-1 (SH-PTP-1)(C-19) (rabbit polyclonal) | Santa Cruz | SC-287 | IB: 1:1000 (7 µL) |
Antibody | Anti-human GAPDH (0411) (mouse monoclonal) | Santa Cruz | SC-47724 | IB: 1:1000 (7 µL) |
Antibody | Anti-human pSHP-1 (S591) (rabbit polyclonal) | ECM Biosciences | Sp-1531 | IB: 1:1000 (7 µL)IF: 1:500 (0.6 µL) |
Antibody | Anti-human pPLCγ (Y783) (rabbit polyclonal) | Cell Signaling | CST-2821S | IB: 1:1000 (7 µL)IF: 1:500 (0.6 µL) |
Antibody | Anti-human pVAV1 (Y160) (rabbit polyclonal) | Bio Source | Bs-44482 | IB: 1:1000 (7 µL)IF: 1:500 (0.6 µL) |
Antibody | Anti-human-PKC-θ (1C2) (mouse monoclonal) | Santa Cruz | SC-81534 | IB: 1:500 (14 µL)IF: 1:250 (1.2 µL) |
Antibody | Goat anti-mouse | Jackson Laboratory | #115-035-003 | 1:10,000 (1 µL) |
Antibody | Goat anti-rabbit | Santa Cruz | Sc-2004 | 1:10,000 (1 µL) |
Antibody | Anti-human KIR2DL1/S1-PE conjugated (mouse monoclonal) | Miltenyi Biotec | 130-099-209 | 1:10 (10 µL) |
Antibody | Anti-human CD107a (LAMP-1) (mouse monoclonal) | BioLegend | #328602 | 1:20 (2.5 µL) |
Antibody | Alexa Fluor-conjugated 488 (goat polyclonal) anti-rabbit IgG (H+L) Highly Cross-Adsorbed Secondary Antibody | Invitrogen | A11034 | IF: 1:2000 (1 µL) |
Antibody | Alexa Fluor-conjugated 488 goat polyclonal anti-mouse IgG (H+L) | Jackson Laboratory | 115-545-146 | IF: 1:2000 (1 µL) |
Recombinant DNA reagent | YFP-SHP-1-CFP (plasmid) | Matalon et al., 2018 | ||
Recombinant DNA reagent | CRISPR CAS9 SHP-1 S591D | Ben-Shmuel et al., 2021 | Backbone pSpCas9 (BB)–2A-GFP vector Addgene plasmid #48138 | |
Sequence-based reagent | siRNA: target PRKCQ gene | Sigma-Aldrich | 5′ CUCUUCACCUGGGCGCCAA 3′5′ UUGGCGCCCAGGUGAAGAG 3′ | |
Sequence-based reagent | siRNA: nonspecific target | Sigma-Aldrich | 5′ UAGCGACUAAACACAUCAA 3′,5′UAAGGCUAUGAAGAGAUAC3′, 5′AUGUAUUGGCCUGUAUUAG3′, 5′ AUGAACGUGAAUUGCUCAA 3′, and 5′ UGGUUUACAUGUCGACUAA3′ | |
Chemical compound, drug | Fluo-3-AM | Biotium | 50016 | 1 µg per sample |
Chemical compound, drug | p-Nitophenyl phosphate (pNPP) | NEB-P0757S | ||
Chemical compound, drug | [35S]Met | PerkinElmer | NEG009L005MC | |
Chemical compound, drug | Monensin | BioLegend | #420701 | |
Chemical reagent | Mirus | Ingenio Solution | MIR50111 | |
Chemical compound, reagent | Enhanced chemiluminescence | PerkinElmer, Life Gene | NEL105001EA,AC2103 | |
chemical compound, reagent | Protein A/G plus-Agarose beads | Santa Cruz Biotechnology | SC-2003 | |
Commercial assay or kit | NK Cell enrichment kit | STEMCELL Technologies | Cat# 19055 | |
Commercial assay or kit | Human PE selection kit | STEMCELL Technologies | Cat# 18551 | |
Software, algorithm | GraphPad Prism | V9.0.1 | RRID:SCR_002798 | |
Software, algorithm | Adobe Photoshop | CC2019 | ||
Software, algorithm | FACS Diva | |||
Software, algorithm | ImageJ 1.53c | https://imagej.nih.gov/ | RRID:SCR_003070 | |
Cell line (Homo sapiens) | YTS KIR2DL1 | RRID:CVCL_D324;parent: CVCL_1797 (YT); DSMZ no. ACC-434 | Kind gift from Prof. Ofer MandelboimSTR profiling testing was done by DSMZ | |
Cell line (H. sapiens) | 721.221 HLA-Cw4 | RRID:CVCL_6263;ATCC: CRL-1855 | Kind gift from Prof. Ofer MandelboimSTR profiling was done by ATCC | |
Cell line (H. sapiens) | 721.221 HLA-Cw7 | RRID:CVCL_6263;ATCC: CRL-1855 | Kind gift from Prof. Ofer MandelboimSTR profiling was done by ATCC | |
Cell line (H. sapiens) | K562 | RRID:CVCL_0004;DSMZ no. ACC-434 | Kind gift from Prof. Ofer MandelboimSTR profiling testing was done by DSMZ | |
Biological sample (H. sapiens) | PBMCs | Blood samples from healthy donors were provided by Magen David Adom (MDA; Israeli National Blood Bank)Donor’s identification information remained anonymous | ||
Strain, strain background (Mus musculus, female) | NOD-Rag1nullIL2rgnull (RAG) | Jackson Labs | NOD-Rag1nullIL2rgnull (RAG) | Details are listed in In vivo conditions and study design table |
Strain, strain background (M. musculus, female) | NOD-SCID IL2Rgammanull (NSG) | Jackson Labs | NOD-SCID IL2Rgammanull (NSG) | Details are listed in In vivo conditions and study design table |
Cell lines and reagents
Request a detailed protocolThe following cells were used in this study: YTS NK cell line expressing the inhibitory KIR2DL1 (referred as YTS-2DL1), B-cell lymphoma, 721.221 cells (referred as 721) expressing no HLA, and 721 cells expressing either HLA-Cw4 or -Cw7, and K562. These cells were kindly provided by Prof. Ofer Mandelboim (Department of Microbiology and Immunology, Faculty of Medicine, Hebrew University of Jerusalem, Israel). All the cell lines were tested negative for mycoplasma. YTS cells were cultured in Iscove’s medium supplemented with 10% fetal bovine serum (FBS), 2 mM L-glutamine, 50 μg/mL penicillin, 50 μg/mL streptomycin, and 50 μM 2-mercapto-ethanol. 721.221 and K562 cells were cultured in RPMI supplemented with 10% FBS, 2 mM L-glutamine, 50 μg/mL penicillin, 50 μg/mL streptomycin, 1% non-essential amino acids, and 1% sodium pyruvate.
Antibodies
Antibodies and their sources were as follows: mouse anti-PLCγ1 (Upstate), mouse anti-VAV1 (D7), rabbit anti-SHP-1 and mouse anti-GAPDH (Santa Cruz), rabbit anti-pSHP-1 (S591) (ECM Biosciences), rabbit anti-pPLCγ1Y783 (Bio Source), rabbit anti-pVAV-1Y160 (Bio Source), and mouse anti-PKC-θ (Santa Cruz). Secondary antibodies: goat anti-mouse (Sigma-Aldrich), goat anti-rabbit (Santa Cruz).
Peripheral blood mononuclear cell (PBMC) isolation
Request a detailed protocolHuman primary PBMCs were isolated from whole blood of healthy donors, as previously described (Barda-Saad et al., 2005). Blood samples from anonymous healthy donors were provided by Magen David Adom (MDA; Israeli National Blood Bank). Informed consent was obtained from all donors. The experiments conformed to the principles set out in the WMA Declaration of Helsinki and the Department of Health and Human Services Belmont Report. The research was performed with approval of and according to the guidelines of the Bar-Ilan University Ethics Committee. Briefly, human PBMCs were isolated from whole blood by Ficoll-Histopaque density gradient centrifugation (MP Biomedical).
Primary NK cells
Request a detailed protocolPrimary NK cells were isolated from PBMCs of healthy donors using the EasySep human NK Cell enrichment kit (STEMCELL Technologies). Subsequently, KIR2DL1-expressing cells were isolated by staining the entire NK cell population with anti-KIR2DL1/S1-PE antibody (Miltenyi Biotec) followed by magnetic separation using the EasySep human PE selection kit (STEMCELL Technologies) according to the manufacturer’s instructions. NK cell isolation efficiencies were >95%. The NK cells were plated in 96-well U-bottomed plates and grown in the presence of irradiated PBLs from two donors (5 × 104 cells from each donor per well) as feeder cells. Cells were expanded in a complete medium containing 1 µg/mL of PHA and 400 U/mL rhuIL-2 (ProSpec). Before experiments, cells were washed to remove the PHA and IL-2, and cultured 48 hr in 60% Dulbecco’s modified Eagle’s medium and 25% F-12 medium supplemented with 10% human serum, 2 mM L-glutamine, 50 μg/mL penicillin, 50 μg/mL streptomycin, 1% non-essential amino acids, and 1% sodium pyruvate.
CRISPR/CAS9-mediated gene knockdown and S591D point mutation
Request a detailed protocolCRISPR/CAS9 knockdown of PTPN6 in YTS cells was conducted according to published protocol and was performed as described in detail (Ran et al., 2013; Matalon et al., 2018) .The pSpCas9(BB)–2A-GFP (PX458) vector was purchased from Addgene (plasmid# 48138). RNA guide sequences targeted to the SHP-1 locus and for knock-in of the S591D mutation were constructed using an online CRISPR design tool (Zhang Lab).
RNA interference
Request a detailed protocolsiRNA to human 3′ UTR PRKCQ was purchased from Sigma-Aldrich. YTS-2DL1 cells were transfected with siRNA specific for the indicated genes or NS siRNA as a control using an AMAXA electroporator.
For knockdown of PRKCQ gene expression, pools of independent specific siRNA oligonucleotides were as follows:
5′ CUCUUCACCUGGGCGCCAA 3′
5′ UUGGCGCCCAGGUGAAGAG 3′
NS siRNA: Pools of nontargeting (nonspecific), negative control siRNA duplexes were obtained from Dharmacon with the following sequences: 5′ UAGCGACUAAACACAUCAA 3′, 5′ UAAGGCUAUGAAGAGAUAC 3′, 5′ AUGUAUUGGCCUGUAUUAG 3′,5′ AUGAACGUGAAUUGCUCAA 3′, and 5′ UGGUUUACAUGUCGACUAA 3′.
Cellular imaging by confocal microscopy
Request a detailed protocolDynamic fluorescence and differential interference contrast (DIC) microscopy images of NK-target conjugates were collected using a Zeiss 510 Meta confocal microscope. All images were collected using a 63× Plan-Apochromat objective (Carl Zeiss).
Chambered cover slips (LabTek) were cleaned by treatment with 1 M HCl, 70% ethanol for 30 min, and dried at 60°C for 30 min. The chambers were treated with a 0.01% (wt/vol) poly-L-lysine solution (Sigma) for 5 min, drained, and dried at 60°C for 30 min.
For NK-target conjugation assays, 5 × 105 target cells were seeded over the bottom of the chamber in 300 μL Optimem medium for 2 hr at 37°C, after which nonadherent cells were rinsed. Then, 5 × 105 NK cells were seeded over the chambers, containing imaging buffer (RPMI medium with 25 mM HEPES without phenol red or serum), and allowed to form conjugates with the target cells for the indicated times at 37°C. Following activation, the cells were fixed for 30 min with 2.5% paraformaldehyde and washed twice with PBS. The NK and target cells in the conjugates were distinguished according to fluorescence signal, with the target cells expressing mCherry. For evaluation of phosphoprotein accumulation at the NKIS, cells were permeabilized with 0.1% Triton X-100 for 5 min. Cells were then blocked for 1 hr in PFN buffer (PBS without Ca2+ and Mg2+ and containing 10% FBS and 0.02% azide) with 2% normal goat serum (Jackson ImmunoResearch). Cells were incubated for 1 hr with the appropriate primary antibodies diluted in blocking medium, followed by staining with isotype-specific, Alexa Fluor-conjugated antibodies for 30 min. Cells were washed three times with PFN between steps. The relative fluorescence intensities of the proteins at the NKIS were determined by measuring the ratio between the fluorescence intensity at the NKIS relative to that at a non-NKIS site using ImageJ software.
Image processing and quantification
Request a detailed protocolThe acquired images were extracted with the LSM browser (Carl Zeiss), cropped, and composed into figures using Adobe Photoshop CC2019.
FRET analysis
Request a detailed protocolFRET was measured by the donor-sensitized acceptor fluorescence technique as previously described (Barda-Saad et al., 2005; Pauker et al., 2012; Fried et al., 2014). Briefly, three sets of filters were used: one optimized for donor fluorescence (excitation, 458 nm; emission, 465–510 nm), a second for acceptor fluorescence (excitation, 514 nm; emission, 530–600 nm), and a third for FRET (excitation, 458 nm; emission, 530–600 nm).
FRET correction
Request a detailed protocolFRET correction was performed as described in detail (Barda-Saad et al., 2005; Pauker et al., 2012; Fried et al., 2014). The non-FRET components were calculated and removed using calibration curves derived from images of single-labeled CFP- or YFP-expressing cells. Sets of reference images were obtained using the same acquisition parameters as those used for the experimental FRET images. To correct for CFP ‘bleed through,’ the intensity of each pixel in the CFP image from CFP-expressing cells was compared to the equivalent pixel in the FRET image of the same cells. A calibration curve was derived to define the level of CFP fluorescence seen in the FRET image as a function of the fluorescence in the CFP image. A similar calibration curve was obtained defining the amount of YFP fluorescence appearing in the FRET image as a function of the intensity in the YFP image using images of cells expressing only YFP. Separate calibration curves were derived for each set of acquisition parameters used in the FRET experiments. Then, using the appropriate calibration curves, together with the CFP and YFP images, the amount of CFP bleed through and YFP cross-excitation was calculated for each pixel in the experimental FRET images. These non-FRET components were subtracted from the raw FRET images, yielding corrected FRET images.
FRET efficiency calculation
Request a detailed protocolThe FRET efficiency (FRETeff) was calculated on a pixel-by-pixel basis using the following equation: FRETeff = FRETcorr/(FRETcorr+ CFP) × 100%, where FRETcorr is the pixel intensity in the corrected FRET image, and CFP is the intensity of the corresponding pixel in the CFP channel image.
To increase the reliability of the calculations and prevent low-level noise from distorting the calculated ratio, we excluded pixels below 50 intensity units and saturated pixels from the calculations and set their intensities to zero. These pixels are shown in black in the ‘pseudocolored’ FRET efficiency images.
To estimate the significance of the FRET efficiency values obtained and exclude the possibility of false-positive FRET results, we prepared cells expressing free CFP and free YFP as negative controls. The FRET efficiency in the negative control system was measured and calculated in the same way as in the main experiment. FRET efficiency values obtained from the negative control samples were subtracted from the values obtained in the main experiments. Image processing and measurements were performed using IPLab software version 3.9.
PTP assay
Request a detailed protocolSHP-1 catalytic activity was determined by measuring the hydrolysis of the exogenous substrate p-nitrophenyl phosphate (pNPP) by SHP-1, as previously described (Lorenz, 2011; Matalon et al., 2018). NK cells (2–5 × 106) were incubated with target cells at a ratio of 1:1 at 37°C for 5 min before lysis. Cells were lysed with ice-cold passive lysis buffer (1.25% Brij, 0.625% n-octyl-β-d-glucoside, 31.3 mM Tris–HCl, pH 7.4, 150 mM NaCl, 6.25 mM ethylenediaminetetraacetic acid, and cOmplete Protease Inhibitor Tablets [Roche]). Cell lysates were subjected to IP (immunoprecipitated) with anti-SHP-1 antibody. Immunoprecipitates were washed twice with ice-cold passive washing buffer (0.1% Brij, 50 mM Tris–HCl, pH 7.4, 300 mM NaCl, and 3.75 mM ethylenediaminetetraacetic acid), and three times with phosphatase buffer (150 mM NaCl, 50 mM HEPES, 10 mM ethylenediaminetetraacetic acid, and 1 mM DDT). Immunoprecipitates were resuspended in 200 µL 25 mM pNPP in phosphatase buffer, and incubated for 30 min at 37°C. Reactions were terminated by adding 800 µL 1 M NaOH, and SHP-1 activity was determined by measuring absorbance at 405 nm.
Cytotoxicity assay
Request a detailed protocolThe cytolytic activity of NK cells against target cells was determined with a standard [35S]Met release assay. Target cells were labeled with [35S]Met (0.2 mCi/mL) for 12–16 hr and washed two times, and then 5 × 103 cells were mixed with NK cells at an effector-to-target ratio of 10:1. Cells were then incubated for 5 hr at 37°C in complete medium. The cells were centrifuged at 200 × g for 5 min, the supernatant was mixed with scintillation liquid, and radioactive signal was measured with a β counter (Packard). Spontaneous release of [35S]Met from an equal number of target cells was determined by adding 100 mL of complete medium to target cells that were incubated without NK cells. Maximal release was determined by adding 100 mL of 0.1 M NaOH to an equal number of target cells in the absence of NK cells. Finally, the percentage of cell lysis caused by the NK cells was calculated using the following equation: % specific lysis = [(sample signal – spontaneous release)/(maximal release – spontaneous release)] × 100.
DNA constructs and mutagenesis
Request a detailed protocolHuman SHP-1 wt cDNA was obtained from Addgene. The cDNA of SHP-1 was subcloned into the expression vector pEYFP-N1 (Clontech) to obtain the chimeric protein YFP-SHP-1; pECFP-N1 (Clontech) was subcloned into the YFP-SHP-1 expression vector to obtain the chimeric protein YFP-SHP-1-CFP. To avoid localization of SHP-1 to the nucleus, YFP-SHP1-CFP was mutated at its NLS sequence (He et al., 2005). Molecular mutants were prepared using the QuikChange II XL site-directed mutagenesis kit (Stratagene). The mCherry plasmid was previously described (Pauker et al., 2012).
Cell transfection and FACS analysis
Request a detailed protocolYTS-2DL1 or 721.221/K562 cells were transfected with Nucleofector 2b (Lonza) using Amaxa solution R and protocol X-001. Transiently transfected cells were used after 24–48 hr. Cells transiently expressing chimeric proteins were selected in hygromycin. Fluorescence analysis and cell sorting were performed using FACSAria or FACSVantage (Becton Dickinson Biosciences).
Cell stimulation, immunoblotting, and immunoprecipitation
Request a detailed protocolFirst, NK cells (primary or YTS cell line) and 721.221 target cells (either expressing HLA-Cw4, Cw7, or no HLA) were incubated separately on ice for 10 min at a ratio of 1:1. The cells were mixed, centrifuged, and incubated on ice for 15 min. The cell mixture was then transferred to 37°C for the indicated period of time and subsequently lysed with ice-cold lysis buffer (1% Brij, 1% n-octyl-β-d-glucoside, 50 mM Tris–HCl, pH 7.6, 150 mM NaCl, 5 mM ethylenediaminetetraacetic acid, 1 mM Na3VO4, and complete Protease Inhibitor Tablets[Roche]). YTS-2DL1 cells were incubated for 30 min on ice with the indicated concentration of pervanadate, before and at the time of NK-target cell co-culture.
For analysis of whole-cell lysates (WCL), 1–5 × 105 cells were used, and for IP experiments 10–15 × 106 cells were used. Protein A/G plus-Agarose beads (Santa Cruz Biotechnology) were used for IP. Protein samples were resolved with sodium dodecyl sulfate-polyacrylamide gel electrophoresis (SDS-PAGE), transferred to nitrocellulose membrane, and immunoblotted with the appropriate primary antibodies. Immunoreactive proteins were detected with either anti-mouse or anti-rabbit horseradish peroxidase-coupled secondary antibody followed by detection with enhanced chemiluminescence (PerkinElmer).
For WCL samples, the phosphorylation or expression level of proteins was measured by densitometric analysis relative to the GAPDH loading control using ImageJ. For IP samples, the relative binding or phosphorylation level was measured by densitometric analysis relative to the precipitation control, using ImageJ.
Measurement of intracellular calcium concentration
Request a detailed protocolFirst, 0.5–1 × 106 NK cells were incubated with 1 µg calcium-sensitive dye, Fluo-3-AM per sample in (MPB) RPMI 1640 medium without phenol red, and containing 0.5 mM probenecid at 37°C for 45 min. The cells were washed once, resuspended in RPMI 1640 without phenol red containing 10 mM HEPES and 0.5 mM probenecid, and maintained at room temperature for 20 min. The cells were incubated at 37°C for 5 min before measurements, then mixed 1:1 with 721 target cells, and the Ca2+ influx was measured by spectrofluorimetry using the Synergy 4 Microplate Reader (BioTek).
CD107a degranulation assay
Request a detailed protocolNK cells (3 × 105) were co-incubated with 6 × 105 target cells expressing mCherry at 37°C for 2 hr in the presence of 2 µM monensin (BioLegend). The cells were centrifuged, incubated with 1:1000 diluted anti-CD107a for 30 min on ice, and washed twice. Cells were then stained with isotype-specific Alexa Fluor-conjugated antibody on ice for 30 min. Cells were washed twice and analyzed by FACS. YTS-2DL1 or pNK-2DL1 cells were distinguished from the target cells based on mCherry expression by the targets.
Xenograft mice model
Request a detailed protocolIn vivo conditions and study design | |
---|---|
Mouse model | Female SCID-NSG or SCID-NRG mice |
Cell type and model | 721.221-cw4 or cw7- B-NHL cell lineSubcutaneous (SC) |
Number of positions injected within the mouse body | 1 |
Number of mice per cage | Five mice per cage |
Irradiation | YTS cells were irradiated for a total of 200 cGy (no irradiation of mice) |
Treatment: intratumor administration | 100 μL of 5 × 106 cells in PBS |
Treatment injection interval | Every 3 days |
Homing | SCID/NOD NSG or NRG female, 6–8-week-old mice were purchased from Envigo or Jackson laboratories. All mice were housed in IVC caging, supplied with irradiated shredded corn cob bedding and irradiated mouse feed diet. The light-dark cycle was 12 hr. The ambient temperature of each room was set at 20°C ± 1 °C. The temperature inside the boxes generally remained between 22°C and 24 °C. Humidity was set between 35% and 55%. |
Ethics oversight | Housing and breeding of mice and experimental procedures were performed according to the guidelines of the Bar-Ilan University Animal Ethics Committee (#82-10-18). |
Study design | Randomization was used to divide the animals for in vivo treatments. No blinding was implemented as the researcher who performed the experiments also performed the analyses. |
NOD-Rag1nullIL2rgnull (RAG) mice and NOD-SCID IL2Rgammanull (NSG) mice were purchased from the Jackson Labs. All mice used were from colonies that were inbred and maintained under SPF conditions at the Bar-Ilan animal house. Housing and breeding of mice and experimental procedures were performed according to the guidelines of the Bar-Ilan University Animal Ethics Committee, and 6–8-week old female RAG mice were subcutaneously injected, between the shoulders, with 3 × 106 721-Cw4 or Cw7 HLA-expressing tumor cells, in 0.1 mL of PBS and Matrigel (Corning) (1:1 ratio). Mice were inspected daily for general well-being, and at the first indication of morbidity (weight loss, lethargy, ruffled fur), or when they reached 8 weeks following inoculation, they were euthanized by CO2. Mice tumor diameters were measured daily with a digital caliper. Tumor volumes were calculated according to the formula:
When the tumor volumes reached 65–75 mm3, mice were intratumorally administered either 5 * 106 irradiated W.T YTS-2DL1 or SHP-1-KO NK cells that were untreated or treated with PKC-θ siRNA. Intratumor injections of NK cells were repeated every 3 days for six injections in total.
Average growth rates of the tumor from initiation of treatment were calculated according to the formula:
Statistical analyses
Request a detailed protocolData calculations of mean ± SEM were conducted in Microsoft Excel (v14.7.2), while data were graphed and statistical analysis was performed using GraphPad Prism 9.0.1 (GraphPad Software, Inc, USA). p-Values were calculated using a two-tailed unpaired t-test or one-sample t-test. Where more than two conditions were compared, a one-way ANOVA or two-way ANOVA with a Tukey post-test was used to calculate p-values. Data are depicted as columns with SE. Statistical parameters and biological replicates are reported in the figure legends.
Data availability
Source data files for numerical data and representative blots are now provided for figures and figure supplements.
References
-
Protein kinase C-theta is required for NK cell activation and in vivo control of tumor progressionJournal of Immunology (Baltimore, Md 182:1972–1981.https://doi.org/10.4049/jimmunol.0801820
-
Comparative analysis of human NK cell activation induced by NKG2D and natural cytotoxicity receptorsEuropean Journal of Immunology 34:961–971.https://doi.org/10.1002/eji.200324705
-
Myosin IIA is required for cytolytic granule exocytosis in human NK cellsThe Journal of Experimental Medicine 204:2285–2291.https://doi.org/10.1084/jem.20071143
-
Modulation of the intracellular inhibitory checkpoint SHP-1 enhances the antitumor activity of engineered NK cellsCellular & Molecular Immunology 18:1314–1316.https://doi.org/10.1038/s41423-020-0443-6
-
Regulation of Src homology 2-containing tyrosine phosphatase 1 during activation of human neutrophils. Role of protein kinase CThe Journal of Biological Chemistry 272:875–882.https://doi.org/10.1074/jbc.272.2.875
-
Specific engagement of the CD94/NKG2-A killer inhibitory receptor by the HLA-E class Ib molecule induces SHP-1 phosphatase recruitment to tyrosine-phosphorylated NKG2-A: evidence for receptor function in heterologous transfectantsEuropean Journal of Immunology 28:1280–1291.https://doi.org/10.1002/(SICI)1521-4141(199804)28:04<1280::AID-IMMU1280>3.0.CO;2-O
-
Protein kinase C-theta inhibitors: A novel therapy for inflammatory disordersCurrent Pharmaceutical Design 18:4725–4746.https://doi.org/10.2174/138161212802651625
-
The Src homology 2 domain tyrosine phosphatases SHP-1 and SHP-2: diversified control of cell growth, inflammation, and injuryHistology and Histopathology 22:1251–1267.
-
Selective protein kinase Cθ (PKCθ) inhibitors for the treatment of autoimmune diseasesBiochemical Society Transactions 42:1524–1528.https://doi.org/10.1042/BST20140167
-
Microchip-Based Single-Cell Imaging Reveals That CD56dimCD57-KIR-NKG2A+ NK Cells Have More Dynamic Migration Associated with Increased Target Cell Conjugation and Probability of Killing Compared to CD56dimCD57-KIR-NKG2A- NK CellsJournal of Immunology (Baltimore, Md 195:3374–3381.https://doi.org/10.4049/jimmunol.1500171
-
Protein kinase C theta (PKCtheta): a key player in T cell life and deathPharmacological Research 55:537–544.https://doi.org/10.1016/j.phrs.2007.04.009
-
EGF-stimulation activates the nuclear localization signal of SHP-1Journal of Cellular Biochemistry 94:944–953.https://doi.org/10.1002/jcb.20307
-
NK cells converge lytic granules to promote cytotoxicity and prevent bystander killingThe Journal of Cell Biology 215:875–889.https://doi.org/10.1083/jcb.201604136
-
Regulation of SHP-1 tyrosine phosphatase in human platelets by serine phosphorylation at its C terminusThe Journal of Biological Chemistry 279:40475–40483.https://doi.org/10.1074/jbc.M402970200
-
Natural killer cell receptor signalingCurrent Opinion in Immunology 15:308–314.https://doi.org/10.1016/s0952-7915(03)00039-6
-
NK cell recognitionAnnual Review of Immunology 23:225–274.https://doi.org/10.1146/annurev.immunol.23.021704.115526
-
Molecular basis of T cell inactivation by CTLA-4Science (New York, N.Y.) 282:2263–2266.https://doi.org/10.1126/science.282.5397.2263
-
Conversion of serine to aspartate imitates phosphorylation-induced changes in the structure and function of microtubule-associated protein tauThe Journal of Biological Chemistry 272:8441–8446.https://doi.org/10.1074/jbc.272.13.8441
-
Rapid T cell receptor-mediated SHP-1 S591 phosphorylation regulates SHP-1 cellular localization and phosphatase activityJournal of Leukocyte Biology 82:742–751.https://doi.org/10.1189/jlb.1206736
-
Negative signaling by inhibitory receptors: the NK cell paradigmImmunological Reviews 224:70–84.https://doi.org/10.1111/j.1600-065X.2008.00660.x
-
SHP-1 and SHP-2 in T cells: two phosphatases functioning at many levelsImmunological Reviews 228:342–359.https://doi.org/10.1111/j.1600-065X.2008.00760.x
-
Protein tyrosine phosphatase assaysCurrent Protocols in Immunology Chapter 11:im1107s93.https://doi.org/10.1002/0471142735.im1107s93
-
NK cells: immune cross-talk and therapeutic implicationsImmunotherapy 3:1143–1166.https://doi.org/10.2217/imt.11.102
-
Protein kinase C-θ clustering at immunological synapses amplifies effector responses in NK cellsJournal of Immunology (Baltimore, Md 189:4859–4869.https://doi.org/10.4049/jimmunol.1200825
-
Defective actin accumulation impairs human natural killer cell function in patients with dedicator of cytokinesis 8 deficiencyThe Journal of Allergy and Clinical Immunology 131:840–848.https://doi.org/10.1016/j.jaci.2012.12.1568
-
Human natural killer cells: origin, clonality, specificity, and receptorsAdvances in Immunology 55:341–380.https://doi.org/10.1016/s0065-2776(08)60513-1
-
Human histocompatibility leukocyte antigen (HLA)-G molecules inhibit NKAT3 expressing natural killer cellsThe Journal of Experimental Medicine 185:385–391.https://doi.org/10.1084/jem.185.3.385
-
Termination of the Activating NK Cell Immunological Synapse Is an Active and Regulated ProcessJournal of Immunology (Baltimore, Md 199:2528–2535.https://doi.org/10.4049/jimmunol.1700394
-
The Emerging Function of PKCtheta in CancerBiomolecules 11:1–12.https://doi.org/10.3390/biom11020221
-
A new member of the protein kinase C family, nPKC theta, predominantly expressed in skeletal muscleMolecular and Cellular Biology 12:3930–3938.https://doi.org/10.1128/mcb.12.9.3930-3938.1992
-
Delivering the kiss of death: progress on understanding how perforin worksCurrent Opinion in Immunology 19:301–308.https://doi.org/10.1016/j.coi.2007.04.011
-
SHP-2 expression negatively regulates NK cell functionJournal of Immunology (Baltimore, Md 183:7234–7243.https://doi.org/10.4049/jimmunol.0900088
-
Genome engineering using the CRISPR-Cas9 systemNature Protocols 8:2281–2308.https://doi.org/10.1038/nprot.2013.143
-
Shedding of CD16 disassembles the NK cell immune synapse and boosts serial engagement of target cellsThe Journal of Cell Biology 217:3267–3283.https://doi.org/10.1083/jcb.201712085
-
Vav1 dephosphorylation by the tyrosine phosphatase SHP-1 as a mechanism for inhibition of cellular cytotoxicityMolecular and Cellular Biology 23:6291–6299.https://doi.org/10.1128/MCB.23.17.6291-6299.2003
-
Spatial organization of signal transduction molecules in the NK cell immune synapses during MHC class I-regulated noncytolytic and cytolytic interactionsJournal of Immunology (Baltimore, Md 167:4358–4367.https://doi.org/10.4049/jimmunol.167.8.4358
-
Crystal structure of human protein tyrosine phosphatase SHP-1 in the open conformationJournal of Cellular Biochemistry 112:2062–2071.https://doi.org/10.1002/jcb.23125
-
BTLA is a lymphocyte inhibitory receptor with similarities to CTLA-4 and PD-1Nature Immunology 4:670–679.https://doi.org/10.1038/ni944
-
SHP-1 suppresses cancer cell growth by promoting degradation of JAK kinasesJournal of Cellular Biochemistry 90:1026–1037.https://doi.org/10.1002/jcb.10727
-
Bystander cells enhance NK cytotoxic efficiency by reducing search timeScientific Reports 7:44357.https://doi.org/10.1038/srep44357
Article and author information
Author details
Funding
Bar-Ilan University (United States–Israel Binational Science Foundation (2019211))
- Mira Barda-Saad
The funders had no role in study design, data collection and interpretation, or the decision to submit the work for publication.
Acknowledgements
This research was partially funded by by the United States–Israel Binational Science Foundation (2019211). The authors thank Danielle Keizer and Dr. Itay Lazar from Bar-Ilan University for their technical assistance. We also thank Dr. Michael Milyavsky and Dr. Adi Zipin-Roitman from Tel-Aviv university for generously providing the NOD-SCID IL2Rγnull (NSG) mice, Dr. Jennifer Benichou Israel Cohen from Bar-Ilan University for her help with the statistical analysis, Dr. Liron Miller and Dr. Natalie Landa from the blood bank and transfusion center of Sheba medical center, Israel, for their technical assistance.
Ethics
Blood samples from healthy donors were randomly collected and provided by Magen David Adom. No patient's identification information was provided. The research was performed according to ethical principles for medical research involving human subjects.
Housing and breeding of mice and experimental procedures were performed according to the guidelines of the Bar-Ilan University Ethics committee.
Copyright
© 2022, Ben-Shmuel et al.
This article is distributed under the terms of the Creative Commons Attribution License, which permits unrestricted use and redistribution provided that the original author and source are credited.
Metrics
-
- 2,125
- views
-
- 316
- downloads
-
- 10
- citations
Views, downloads and citations are aggregated across all versions of this paper published by eLife.
Download links
Downloads (link to download the article as PDF)
Open citations (links to open the citations from this article in various online reference manager services)
Cite this article (links to download the citations from this article in formats compatible with various reference manager tools)
Further reading
-
- Immunology and Inflammation
The incidence of metabolic dysfunction-associated steatotic liver disease (MASLD) has been increasing worldwide. Since gut-derived bacterial lipopolysaccharides (LPS) can travel via the portal vein to the liver and play an important role in producing hepatic pathology, it seemed possible that (1) LPS stimulates hepatic cells to accumulate lipid, and (2) inactivating LPS can be preventive. Acyloxyacyl hydrolase (AOAH), the eukaryotic lipase that inactivates LPS and oxidized phospholipids, is produced in the intestine, liver, and other organs. We fed mice either normal chow or a high-fat diet for 28 weeks and found that Aoah-/- mice accumulated more hepatic lipid than did Aoah+/+ mice. In young mice, before increased hepatic fat accumulation was observed, Aoah-/- mouse livers increased their abundance of sterol regulatory element-binding protein 1, and the expression of its target genes that promote fatty acid synthesis. Aoah-/- mice also increased hepatic expression of Cd36 and Fabp3, which mediate fatty acid uptake, and decreased expression of fatty acid-oxidation-related genes Acot2 and Ppara. Our results provide evidence that increasing AOAH abundance in the gut, bloodstream, and/or liver may be an effective strategy for preventing or treating MASLD.
-
- Immunology and Inflammation
- Microbiology and Infectious Disease
Pseudomonas aeruginosa (PA) is an opportunistic, frequently multidrug-resistant pathogen that can cause severe infections in hospitalized patients. Antibodies against the PA virulence factor, PcrV, protect from death and disease in a variety of animal models. However, clinical trials of PcrV-binding antibody-based products have thus far failed to demonstrate benefit. Prior candidates were derivations of antibodies identified using protein-immunized animal systems and required extensive engineering to optimize binding and/or reduce immunogenicity. Of note, PA infections are common in people with cystic fibrosis (pwCF), who are generally believed to mount normal adaptive immune responses. Here, we utilized a tetramer reagent to detect and isolate PcrV-specific B cells in pwCF and, via single-cell sorting and paired-chain sequencing, identified the B cell receptor (BCR) variable region sequences that confer PcrV-specificity. We derived multiple high affinity anti-PcrV monoclonal antibodies (mAbs) from PcrV-specific B cells across three donors, including mAbs that exhibit potent anti-PA activity in a murine pneumonia model. This robust strategy for mAb discovery expands what is known about PA-specific B cells in pwCF and yields novel mAbs with potential for future clinical use.