Dendritic branch structure compartmentalizes voltage-dependent calcium influx in cortical layer 2/3 pyramidal cells
Figures
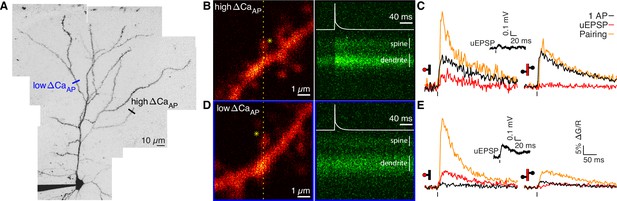
Voltage-dependent calcium influx varies in a branch-specific manner throughout L2/3 pyramidal cells.
(A) Maximum z-projection of Alexa 594 fluorescence showing the full apical dendritic morphology of a cortical L2/3 pyramidal cell. Two sites corresponding to the dendritic regions with low (blue) and high (black) ΔCaAP are indicated. (B) Frame scan (left) showing high ΔCaAP branch. Dotted yellow line indicates the orientation of the line scan used to acquire the data in the kymograph (right) of Fluo-5F fluorescence in the spine and neighboring dendrite evoked by a bAP. Star indicates glutamate uncaging location. White vertical lines indicate ROIs for spine and dendrite. Inset: bAP waveform recorded at the soma. (C) Average calcium-dependent fluorescence transients in a high ΔCaAP dendritic spine (left) and parent dendrite (right) evoked by 1 bAP, an uEPSP, and pairing of an uEPSP with 1 bAP (bAP evoked 5ms after the uEPSP). Inset: average somatic whole-cell recording of the uEPSPs. In this and all presentations of imaging data, the red shaded area of the spine and dendritic schematic indicates the region from which fluorescence was measured. (D) As in B for the low ΔCaAP branch. (E) As in C for the low ΔCaAP branch. Note the difference in ΔCaAP (black) between the spines in B-C and D-E. bAP-evoked calcium influx and bAP-dependent amplification are decoupled in L2/3 pyramidal cells.
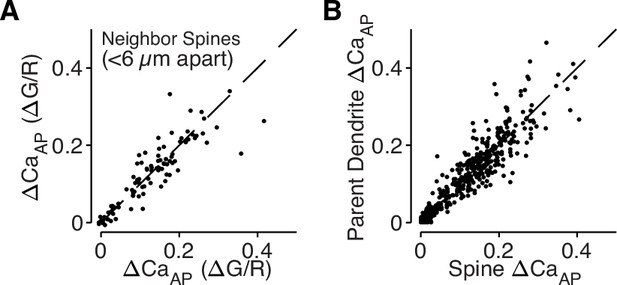
bAP-evoked calcium influx is regulated across dendritic branches and consistent in neighboring dendritic spines.
(A) Comparison of ΔCaAP in neighboring dendritic spines measured simultaneously. The strong correlation indicates that nearby spines have similar calcium signals throughout the dendritic tree and intracellular differences in ΔCaAP are only observed across branches (N = 94, R2 = 0.79). (B) Comparison of ΔCaAP between dendritic spines and their parent dendritic shafts. The strong correlation indicates that ΔCaAP is regulated at the level of dendritic branches and motivates our use of dendritic calcium influx as a proxy for nearby dendritic spines (N = 526, R2 = 0.85).
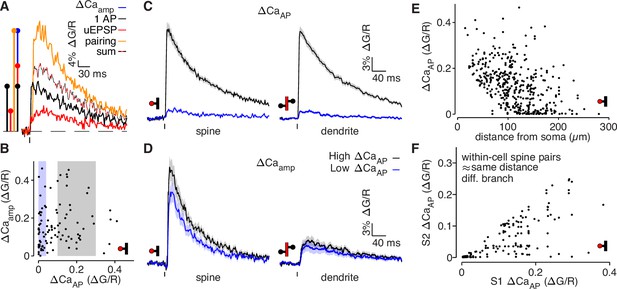
bAP-evoked calcium influx and bAP-dependent amplification are decoupled in L2/3 pyramidal cells.
(A) Average calcium-dependent fluorescence transients recorded from a dendritic spine and a schematic of the method for computing the amplification of calcium influx caused by interaction of the bAP and uEPSP, which results in additional influx (blue) relative to the sum of the bAP and uEPSP components measured independently (black/red). (B) bAP-dependent amplification vs. bAP-evoked calcium influx. Shaded areas indicate selection of high ΔCaAP (gray) and low ΔCaAP (blue) spines used in panels C and D. (C) ΔCaAP in high (black) and low (blue) ΔCaAP dendritic spines (left) and parent dendritic shafts (right). In this and all presentations of average fluorescence transients, the line and shaded areas show the mean and standard error around the mean. (D) Δ Caamp in high (black) and low (blue) ΔCaAP dendritic spines (left) and parent dendritic shafts (right). (E) ΔCaAP across distance from soma in all dendritic spines (N = 526/95/29). (F) Comparison of ΔCaAP between pairs of dendritic spines (i.e. S1 & S2) that were recorded from the same cell, on different dendritic branches, and at a similar distance from the soma ( < 20 µm relative distance from soma). Each pair was sorted so the abscissa indicates the ΔCaAP of the spine with higher calcium influx (N = 94/22/6).
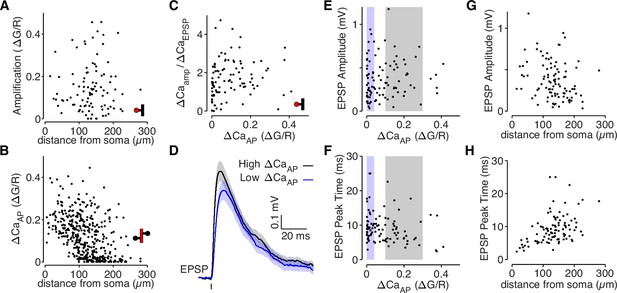
bAP-dependent amplification is not determined by glutamate uncaging properties.
(A) bAP-dependent amplification of uEPSP-evoked calcium influx across distance from soma in all dendritic spines. (B) ΔCaAP across distance from soma in all dendritic shafts (N = 412). (C) Comparison of bAP-dependent amplification divided by calcium evoked by the uEPSP (to normalize for the level of NMDAR activation) with bAP-evoked calcium influx. The plot is qualitatively similar to the plot in Figure 2B, suggesting that differences in NMDAR activation do not explain the results. (D) Average uEPSP recorded from the soma for high (black) and low (blue) ΔCaAP dendritic spines. Selection criterion indicated by black and blue patches in panels E and F. (E) Comparison of uEPSP amplitude with bAP-evoked calcium influx. (F) Comparison of uEPSP peak time with bAP-evoked calcium influx. The small negative correlation is primarily because low ΔCaAP dendritic spines tend to be further from the soma, so the slower uEPSPs are primarily due to cable filtering rather than synaptic properties. (G) Comparison of uEPSP amplitude with distance from soma. (H) Comparison of uEPSP peak time with distance from soma.
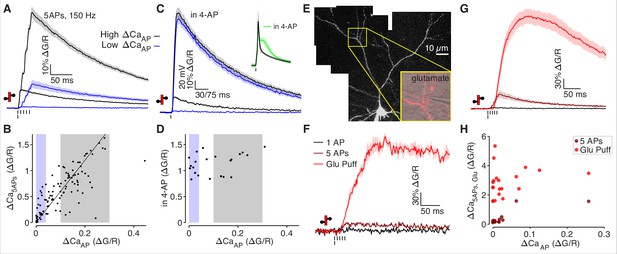
Low ΔCaAP dendrites contain voltage-gated calcium channels.
(A) Calcium-dependent fluorescence transients evoked by 1 bAP or 5 bAPs at 150 Hz in high (black) and low (blue) ΔCaAP dendrites. (B) Comparison of peak calcium influx evoked by 1 bAP or 5 bAPs in each dendrite. Gray and blue patches indicate selection of high (gray) and low (blue) ΔCaAP spines used in panel A. The line y = 6 x is plotted for reference. N = 109/33/9, R2 = 0.609, F = 166, p = 1.5 × 10–23.(C) Calcium-dependent fluorescence transients evoked by 1 bAP before and after application of 2 mM 4-AP in high (black) and low (blue) ΔCaAP branches. Inset: average AP waveform before and after application of 4-AP. (D) Comparison of peak calcium influx evoked by 1 bAP before and after application of 2 mM 4-AP. Gray and blue patches indicate selection of high (gray) and low (blue) ΔCaAP spines used in panel C. There was no significant correlation: N = 22/7/3, R2 = 0.135, F = 3.11, p = 0.093. (E) Maximum z-projection of Alexa 594 fluorescence from a L2/3 pyramidal cell with differential-interference contrast image overlaid showing glutamate puffing pipette. The full apical dendritic morphology was not imaged. (F) Calcium-dependent fluorescence transients evoked by 1 bAP, 5 bAPs at 150 Hz, and a 1 mM glutamate puff in the example branch from panel E. (G) Average calcium-dependent fluorescence transients evoked by 1 bAP, 5 bAPs at 150 Hz, and a 1 mM glutamate puff across all branches recorded. (H) Comparison of peak calcium influx evoked by bAP, 5 bAPs at 150 Hz, and a 1 mM glutamate puff in all branches. The response to 5 bAPs was not recorded in all dendrites (N = 20/6/3).
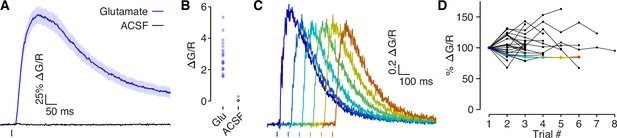
Glutamate puff-evoked calcium signals are mediated by voltage-gated calcium channels.
(A) Dendritic calcium influx evoked by picospritzer puff of either 1 mM glutamate (N = 22) or ACSF (N = 4). (B) Comparison of peak calcium influx evoked by 1 mM glutamate and ACSF. No calcium influx was observed in response to ACSF, indicating that the calcium signal was not due to mechanical displacement evoked by the picospritzer. (C) Example traces of calcium influx evoked by 1 mM glutamate puff for one dendrite across all trials, shifted along the x-axis to visualize each trial clearly. (D) Dendritic calcium influx evoked by 1 mM glutamate for each trial, normalized to first trial amplitude. If glutamate was out-competing CPP (a competitive antagonist) at NMDAR’s binding sites, then the presence of MK-801, a use-dependent blocker, should reduce NMDAR conductance in each trial. No systematic reduction in the calcium signal was observed, indicating that NMDARs were fully blocked. Colored points indicate example used in panel C.
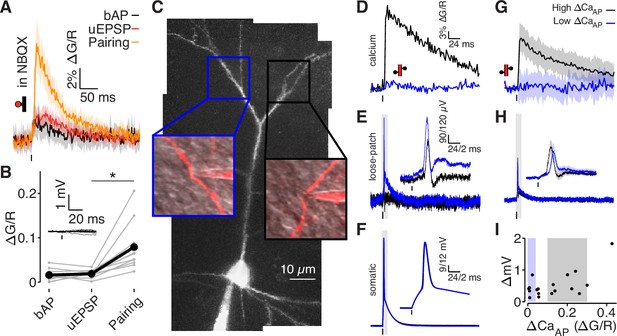
bAPs propagate to low ΔCaAP dendrites in the absence of EPSPs.
(A) Calcium-dependent fluorescence transients evoked by bAPs, uEPSPs, and bAP/uEPSP pairings in the presence of 10 µM NBQX. (B) Comparison of peak calcium influx in spines evoked by bAP, uEPSP, and bAP/uEPSP pairing, in the presence of 10 µM NBQX. Inset: whole-cell recordings from glutamate uncaging in the presence of NBQX. Calcium influx evoked by pairing was significantly higher than by the uEPSP alone, t-test: N = 12/8/2, t = 4.01, p = 5.8 × 10–4. (C) Maximum z-projection of Alexa 594 fluorescence from a L2/3 pyramidal cell with a whole-cell recording in the soma and consecutive loose-patch recordings in two branches. Insets: red fluorescence overlaid on differential interference contrast image of loose-patch configuration for each dendrite. The full apical dendritic morphology was not imaged. (D) Calcium-dependent fluorescence transients evoked by bAPs in each dendrite from panel C, recorded during loose-patch recordings. (E) Electrical signals evoked by bAPs measured with dendritic loose-patch recordings in each dendrite from panel C. Inset: expanded trace from gray shaded region. (F) Somatic whole-cell recording of bAPs during each loose-patch recording from panel C. Inset: expanded trace from gray shaded region. (G) Average calcium-dependent fluorescence transients evoked by bAPs in high (black) and low (blue) ΔCaAP branches during loose-patch recordings. (H) Average electrical signal evoked by bAPs in high (black) and low (blue) ΔCaAP branches measured with loose-patch recordings. (I) Comparison of peak calcium influx and peak electrical signal evoked in each dendrite by bAPs. Patches indicate selection of high (gray) and low (blue) ΔCaAP branches used in panels G and H (N = 19/15/9).
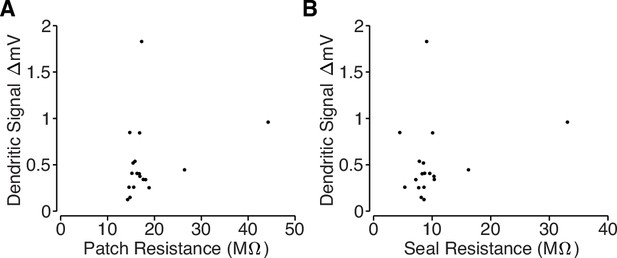
Loose-patch recording properties.
(A) Comparison of total resistance of loose-patch recordings and amplitude of the dendritic electrical signals. The total resistance includes the pipette resistance and the seal resistance, which was measured after acquiring a loose-patch seal on a target dendrite using a small current step. (B) Comparison of the seal resistance and the amplitude of the dendritic electrical signals. Seal resistance was computed by subtracting the loose-patch resistance (measured after acquiring a loose-patch seal) from the pipette resistance (measured with the pipette in the bath solution). Note: several unmeasured noise sources prohibit inference of intracellular bAP amplitude based on loose-patch recordings. We are primarily measuring capacitive currents, , but we cannot directly measure dendritic capacitance, which may differ across dendritic branches. Also, the amplitude of the recording is also proportional to the true seal resistance (Figure 4D); however, our loose-patch recordings used large pipettes (pipette resistance ≈8 MΩ) and the total patch resistance only increased by ∼10 MΩ (Figure 4C), so estimates of the seal resistance are likely to be contaminated by the neuropil.
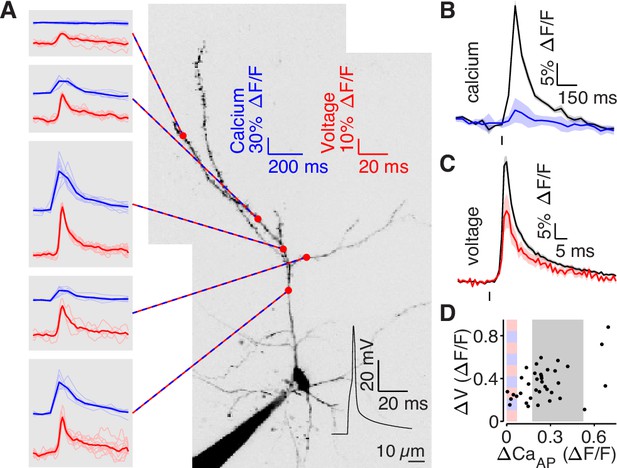
bAP amplitude is attenuated in low branches.
(A) Mean calcium-dependent (blue) and voltage-dependent (red) fluorescence transients evoked by 1 bAP (left) measured in the 5 branches indicated in the maximum z-projection of Alexa 594 fluorescence from a L2/3 pyramidal cell (right).The black trace shows the bAP waveform recorded from the soma during calcium and voltage imaging. Note that calcium- and voltage-dependent transients are shown on ~10 fold different time scales. The full apical dendritic morphology was not imaged. (B) Average calcium-dependent fluorescence transient in high (black) and low (blue) Δ CaAP branches evoked by 1 bAP. (C) Average voltage-dependent fluorescence transient in high (black) and low (red) Δ CaAP branches evoked by 1 bAP. (D) Comparison of the peak voltage- and calcium-dependent fluorescence transient evoked by 1 bAP. Shaded regions indicate selection criterion for high (gray) and low (blue/red) ΔCaAP branches. Calcium-dependent fluorescence predicted voltage-dependent fluorescence, N = 37, R2 = 0.243, F = 11.2, p = 1.9 × 10–3 and the y-intercept was significantly above 0, y-int = 0.235, t(35)=5.34, p = 5.6 × 10–3.
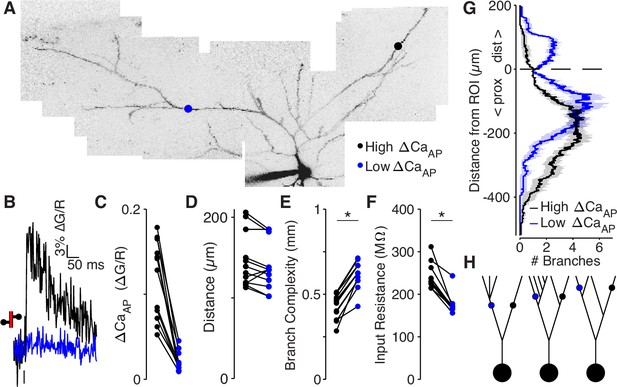
Low ΔCaAP dendrites have a more elaborate branch structure than high ΔCaAP dendrites.
(A) Maximum z-projection of Alexa 594 fluorescence from a L2/3 pyramidal cell. Dots indicate location of calcium imaging sites for a high (black) and low (blue) ΔCaAP branch. The two sites are approximately the same distance from the soma (high ΔCaAP: 145 µm, low ΔCaAP: 131 µm). (B) Calcium-dependent fluorescence transient evoked by bAP in high (black) and low (blue) ΔCaAP sites from panel A. (C) Comparison of ΔCaAP in distance-matched, within-cell pairs. N = 20/8/8, N = 12 pairs, Ratio: 0.17, (range: 0.06–0.29). (D) Comparison of the distances from the soma for each pair of recording sites. (E) Comparison of branch complexity in high (black) and low (blue) ΔCaAP sites, using a distance-discounted measurement of nearby dendritic branches. Branch complexity was significantly higher in low ΔCaAP sites, U-Test, U = 215, z = 3.73, p = 1.9 × 10–4. (F) Comparison of input resistance for high (black) and low (blue) ΔCaAP sites shown in panels C and D, measured in computational simulations of compartment models of each cell in NEURON. Input resistance was significantly lower in low ΔCaAP sites, U-Test, U = 213, z = 3.61, p = 3.1 × 10–4. (G) Average number of branches at a given distance from a recording site for high (black) and low (blue) ΔCaAP dendrites. This curve was multiplied by a symmetric exponential filter with a length constant of 145 µm and then integrated to compute the branch complexity in panel E. Mean ± SEM. (H) Schematic of morphologies with same branch order but different branch complexity. The blue and black site in each neuron have the same branch order, but the branch complexity of the blue site is higher due to the number of branches distal to the site (left), the number of branches on a sister dendrite (middle), and the distance from a previous branch (right).
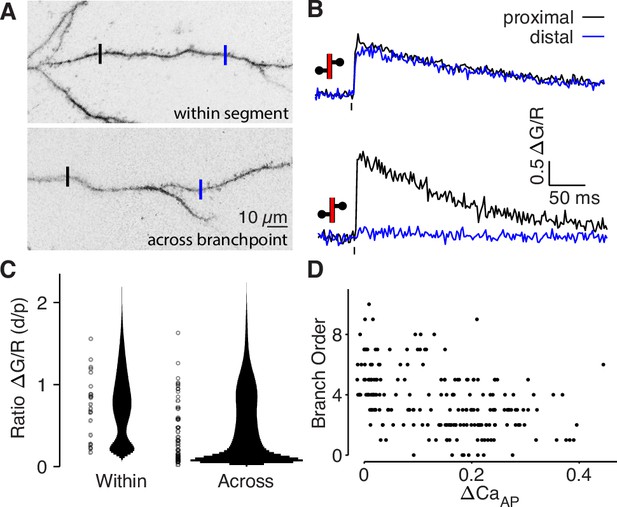
bAP-evoked calcium influx attenuates around branch points.
(A) Alexa 594 MZP showing two ROIs within a segment of dendrite or across a branch point. Blue ROI is more distal than black ROI. (B) Calcium influx evoked by bAP in ROIs from panel A. (C) Distribution of the ratio of peak calcium influx (distal/proximal) for within-segment pairs (N = 22, median = 0.798) and across branchpoint pairs (N = 41, median = 0.361). U-test: U = 874, z = p = 0.015. Width of bar indicates number of observations. (D) Comparison of dendritic bAP-evoked calcium influx and branch order (N = 202, R2 = 0.178, F = 43.3, p = 4 × 10–10).
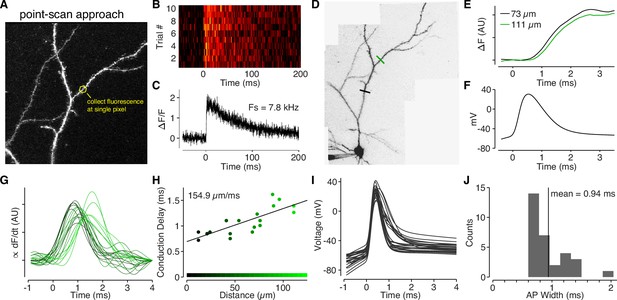
Back-propagating APs span 145 µm in L2/3 cell dendrites.
(A) Alexa 594 MZP showing location of point ROI. The scan mirrors were parked at angle such that excitation light impinged directly on an ROI of interest (in this case a dendritic shaft), and fluorescence was collected at the maximal sample rate of the DAQ board. (B) Calcium-dependent fluorescence transients evoked by a bAP for 10 trials collected at the ROI indicated in panel A. (C) Average ΔF/F for the 10 trials in panel B. (D) Alexa 594 MZP showing locations of two point ROIs that were 73 µm and 111 µm from the soma. (E) Normalized and smoothed for the two ROIs indicated in panel D. Note the small delay for the further ROI. (F) Somatic action-potential waveform recording from the cell in panel D. (G) Smoothed derivative of ΔCaAP in dendritic spines, color-coded by their distance from the soma (color scale in panel H). We used the derivative to accurately measure the time-course of calcium influx in each spine. (H) Conduction delay of ΔCaAP (measured at peak of derivative) as a function of distance. The inverse of the slope gives the conduction velocity of APs as they back-propagate through L2/3 cell dendrites. (I) Waveforms of APs recorded from L2/3 cells aligned to peak of second derivative (kink of AP waveform). (J) Histogram of FWHM of AP waveform for each cell. To compute the spatial spread of APs in L2/3 cell dendrites, we multiplied the average AP width by the conduction velocity .
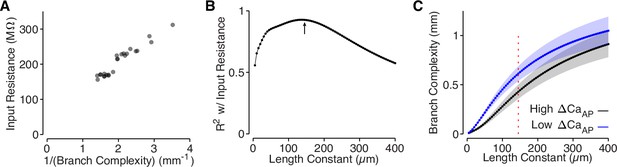
Branch complexity is correlated with input resistance.
(A) Input resistance of each site, measured in NEURON, compared to the inverse of branch complexity of each site, measured based on the cell’s dendritic morphology using a length constant of 145 µm. R2 = 0.93 at λ = 145. We took the inverse because branch complexity should scale linearly with input conductance, which is the inverse of input resistance. (B) Correlation (R2) between branch complexity and input resistance for each length constant used to compute branch complexity. The arrow indicates the length constant used in panel A and Figure 6, computed based on the spatial waveform of the AP in L2/3 cell dendrites. (C) Comparison of branch complexity between high and low ΔCaAP dendrites for each length constant of branch complexity. Red dotted line indicates length constant used in panel A and Figure 6. Y-axis is on a log scale.
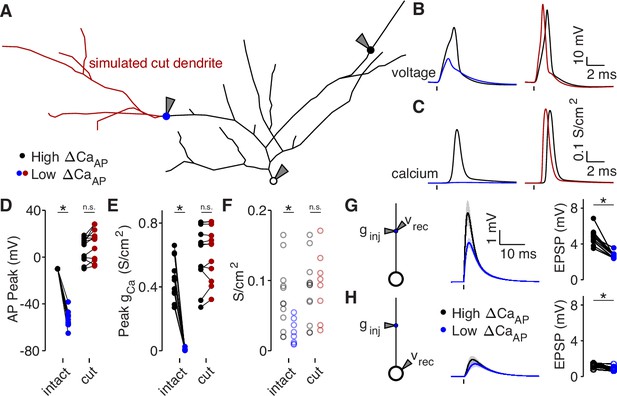
Dendritic branch structure is sufficient to explain reductions in bAP-evoked calcium influx.
(A) Schematic of a neuron morphology used for NEURON simulations derived from the neuron shown in Figure 6A. Blue and black dots indicate high (black) and low (blue) ΔCaAP recording sites. Red dendrites indicate section of dendrite that was computationally ‘cut’ in a subset of experiments. Recordings and injections were performed at the sites indicated with the gray triangles. (B) Dendritic voltage recordings of bAPs from high (black) and low (blue/red) ΔCaAP sites in panel A in NEURON for an intact cell (left) or with cut dendrites (right). (C) Calcium conductances recorded from high (black) and low (blue/red) ΔCaAP sites in panel A evoked by a back-propagating bAP in NEURON for an intact cell (left) or with cut dendrites (right). (D) Comparison of peak bAP voltage for each high/low ΔCaAP pair recorded in NEURON from same sites as experimental recordings, for intact and cut cells (N = 12). Dendritic potassium channel density was fit so the high ΔCaAP site would have a peak of –10 mV in intact cells. AP Peak was significantly reduced in low ΔCaAP sites in the intact cells (U-Test, U = 78, z = 4.13, p = 3.7 × 10–5) but not different in cut cells (U-Test, U = 168, z = 1.01, p = 0.31). (E) Comparison of peak calcium conductance during bAP for each high/low ΔCaAP site pair, as in panel D. Peak calcium conductance was significantly reduced in low ΔCaAP sites in the intact cells (U-Test, U = 78, z = 4.13, p = 3.7 × 10–5) but not different in cut cells (U-Test, U = 155, z = 0.26, p = 0.79). (F) A-Type potassium channel density required for the bAP to peak at –10 mV for each site in intact or cut cells. A-Type potassium channel density was significantly reduced in low ΔCaAP sites in the intact cells (U-Test, U = 160, z = 2.58, p = 9.7 × 10–3) but not different in cut cells (U-Test, U = 120, z = 0.42, p = 0.67). (G) Left: a synaptic conductance was injected into each site and the local EPSP was recorded in NEURON. Middle: dendritic EPSPs recorded in high and low ΔCaAP dendritic sites. Right: comparison of dendritic EPSP amplitudes recorded in each high/low ΔCaAP pair. Mean ± SEM. Dendritic EPSP amplitude was significantly reduced in low ΔCaAP sites (U-Test, U = 79, z = 4.07, p = 4.7 × 10–5). (H) Same as in G, but with synaptic conductance injected into dendrite and recorded in the soma. Somatic EPSP amplitude was significantly reduced in low ΔCaAP sites (U-Test, U = 101, z = 2.8, p = 5.1 × 10–3), but had a smaller effect size than difference in dendritic EPSP amplitude (see text).
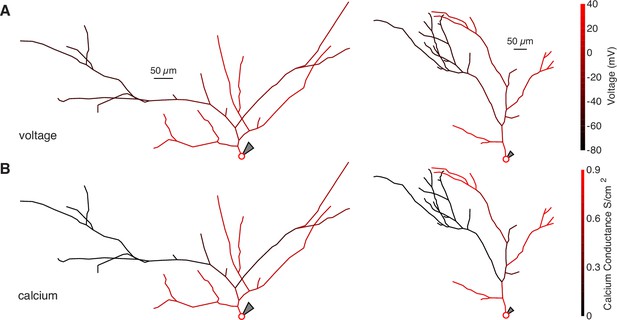
Schematics of bAP amplitude and peak calcium conductance throughout NEURON compartment models.
(A) Schematic of two neurons modeled in NEURON. The color of each segment indicates the peak voltage reached during a back-propagating AP. (B) Same as above but with color indicating peak calcium conductance during a back-propagating AP.
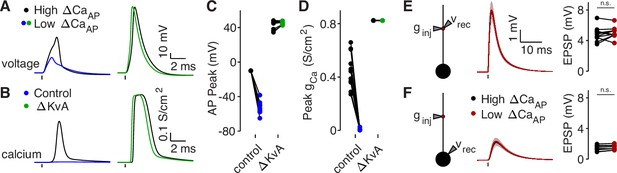
Branch differences are normalized by blocking A-Type potassium channels or cutting dendrites.
(A) Dendritic voltage recordings of bAPs from high (black) and low (blue/green) ΔCaAP sites in panel A in NEURON in a control cell (left) or with A-Type potassium channels removed (right). We removed A-Type potassium channels to simulate the application of 4-AP. (B) Calcium conductances recorded from high (black) and low (blue/green) ΔCaAP sites in panel A evoked by a back-propagating bAP in NEURON in a control cell (left) or with A-Type potassium channels removed (right). (C) Comparison of peak bAP voltage for each high/low ΔCaAP pair recorded in NEURON from same sites as experimental recordings, for control cells and with simulated 4-AP application (N = 12). (D) Comparison of peak calcium conductance during bAP for each high/low ΔCaAP site pair, as in panel C. (E) Same as in Figure 7G, but with cut dendrites. Left: a synaptic conductance was injected into each site and the local EPSP was recorded in NEURON compartment models with cut dendrites. Middle: dendritic EPSPs recorded in high (black) and low (maroon) ΔCaAP dendritic sites. Right: comparison of dendritic EPSP amplitudes recorded in each high/low ΔCaAP pair. Dendritic EPSP amplitude was not different between high and low ΔCaAP sites after simulated cutting (U-Test, U = 161, z = 0.61, p = 0.54). (F) Same as in Figure 7H, but with cut dendrites. Synaptic conductance injected into dendrite and recorded in the soma. Somatic EPSP amplitude was not different between high and low ΔCaAP sites after simulated cutting (U-Test, U = 157, z = 0.38, p = 71).
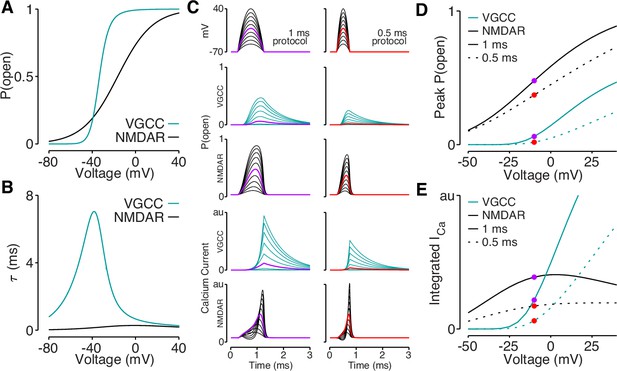
Reductions in bAP amplitude have a selective effect on VGCC-mediated calcium influx.
(A) Steady-state open probability of VGCCs and NMDARs as a function of voltage due to voltage-dependent activation of VGCCs and Mg2+-block of NMDARs. (B) Voltage-dependent time constant of the activation gate for VGCCs and Mg2+ block for NMDARs. (C) We simulated the response of VGCCs and NMDARs to a 1ms (left) or 0.5ms (right) quadratic depolarizing voltage with varying amplitude (1st row) designed to resemble bAPs. Voltage-dependent open-probability (2nd and 3rd rows) and voltage-dependent calcium current (4th and 5th rows) for VGCCs (teal) and NMDARs (black). Purple and red traces correspond to summary data in panels D and E. (D) Peak open probability of VGCCs and NMDARs during quadratic voltage protocols in panel C. (E) Integral of calcium influx through VGCCs and NMDARs during quadratic voltage protocols in panel C.