Drosophila model to clarify the pathological significance of OPA1 in autosomal dominant optic atrophy
eLife assessment
This study provides valuable insights into the complex genetics of dominant optic atrophy. Leveraging a fly model, the investigators provide solid evidence, albeit with small effect sizes, for a dominant negative mechanism of certain pathogenic variants that tend to cause more severe phenotypes, a long held hypothesis in the field. The work is of high interest to those in the optic atrophy and degeneration fields.
https://doi.org/10.7554/eLife.87880.3.sa0Valuable: Findings that have theoretical or practical implications for a subfield
- Landmark
- Fundamental
- Important
- Valuable
- Useful
Solid: Methods, data and analyses broadly support the claims with only minor weaknesses
- Exceptional
- Compelling
- Convincing
- Solid
- Incomplete
- Inadequate
During the peer-review process the editor and reviewers write an eLife Assessment that summarises the significance of the findings reported in the article (on a scale ranging from landmark to useful) and the strength of the evidence (on a scale ranging from exceptional to inadequate). Learn more about eLife Assessments
Abstract
Autosomal dominant optic atrophy (DOA) is a progressive form of blindness caused by degeneration of retinal ganglion cells and their axons, mainly caused by mutations in the OPA1 mitochondrial dynamin like GTPase (OPA1) gene. OPA1 encodes a dynamin-like GTPase present in the mitochondrial inner membrane. When associated with OPA1 mutations, DOA can present not only ocular symptoms but also multi-organ symptoms (DOA plus). DOA plus often results from point mutations in the GTPase domain, which are assumed to have dominant-negative effects. However, the presence of mutations in the GTPase domain does not always result in DOA plus. Therefore, an experimental system to distinguish between DOA and DOA plus is needed. In this study, we found that loss-of-function mutations of the dOPA1 gene in Drosophila can imitate the pathology of optic nerve degeneration observed in DOA. We successfully rescued this degeneration by expressing the human OPA1 (hOPA1) gene, indicating that hOPA1 is functionally interchangeable with dOPA1 in the fly system. However, mutations previously identified did not ameliorate the dOPA1 deficiency phenotype. By expressing both WT and DOA plus mutant hOPA1 forms in the optic nerve of dOPA1 mutants, we observed that DOA plus mutations suppressed the rescue, facilitating the distinction between loss-of-function and dominant-negative mutations in hOPA1. This fly model aids in distinguishing DOA from DOA plus and guides initial hOPA1 mutation treatment strategies.
Introduction
Autosomal dominant optic atrophy (DOA) is a progressive form of blindness characterized by selective degeneration of retinal ganglion cells (RGCs) and the axons that form the optic nerve. Despite being a rare disease with a frequency of 1/12,000 to 1/50,000, DOA is the most commonly diagnosed form of hereditary optic neuropathy. In 2000, OPA1 mitochondrial dynamin like GTPase (OPA1) was identified as the causative gene for DOA (Alexander et al., 2000; Delettre et al., 2000).
OPA1 encodes a dynamin-like GTPase located in the inner mitochondrial membrane (Olichon et al., 2002). It has various functions, including mitochondrial fusion, mitochondrial DNA (mtDNA) maintenance, control of cell death, and resistance to reactive oxygen species (ROS) (Lenaers et al., 2021). The most common pathogenic mutation in OPA1 is the c.2708–2711 delTTAG deletion (Toomes et al., 2001). Five pathogenic mutations, including the one above, result in a substantial decrease in OPA1 protein in the fibroblasts from affected patients (Zanna et al., 2008), supporting the theory that DOA is caused by haploinsufficiency.
Optic atrophy is a characteristic feature of OPA1-associated DOA; however, multisystem symptoms have been reported in up to 20% of OPA1 mutation carriers (Yu-Wai-Man et al., 2010a). This is called DOA plus. DOA plus can include sensorineural deafness (Amati-Bonneau et al., 2008), multiple sclerosis-like illness (Verny et al., 2008; Yu-Wai-Man et al., 2016), parkinsonism and dementia (Carelli et al., 2015; Lynch et al., 2017), and cardiomyopathy (Spiegel et al., 2016). The R445H mutation is a well-known mutation associated with DOA plus (Amati-Bonneau et al., 2008; Amati-Bonneau et al., 2003; Shimizu et al., 2003). The proportion of mutated OPA1 locations differs between patients with DOA and DOA plus. Missense mutations in the dynamin-featured structure, especially the GTPase domain of OPA1, are more likely to cause severe symptoms compared with loss-of-function (LOF) mutations such as deletions or splice site mutations (Yu-Wai-Man et al., 2010a). This is probably due to the dominant-negative (DN) effect of a DOA plus mutation on the normal OPA1 allele, since OPA1 functions as a homo-oligomer (Frezza et al., 2006; Olichon et al., 2006); in fact, its yeast ortholog, Mgm1, forms oligomers, increasing the GTPase activity (Meglei and McQuibban, 2009; Rujiviphat et al., 2009). Particularly, the dynamin-featured structure accounts for 70% of the 233 pathogenic variants of DOA and DOA plus described in the locus-specific database (Ferré et al., 2015). However, mutations in these domains do not determine DOA plus, as it affects only 20% of all OPA1 mutation carriers. Thus, experimental models to determine whether a gene mutation is LOF or DN are required to distinguish between DOA and DOA plus. Although currently there is no effective treatment for DOA or DOA plus, distinguishing between them allows earlier planning of interventions such as hearing aids, rather than relying solely on visual aids, necessary only for those with DOA plus. In the quest to differentiate between LOF and DN effects within the context of genetic mutations, precedents exist in simpler systems such as yeast and human fibroblasts. These models have provided valuable insights into the conserved functions of OPA1 across species, as evidenced by studies in yeast models (Del Dotto et al., 2017) and fibroblasts derived from patients harboring OPA1 mutations (Kane et al., 2017). However, the ability to distinguish between LOF and DN effects in an in vivo model organism, particularly at the structural level of retinal axon degeneration, has remained elusive. This gap underscores the necessity for a more complex model that not only facilitates molecular analysis but also enables the examination of structural changes in axons and mitochondria, akin to those observed in the actual disease state.
As OPA1 is conserved in various species, DOA models have been reported in vertebrates such as mice and zebrafish, simple organisms such as nematodes and fruit flies, and in vitro yeast and cultured cell models (Del Dotto and Carelli, 2021). In mouse models, focal RGC axons decrease in number with mitochondrial abnormalities at the electron microscope level, but the phenotype appears slowly, not allowing for analysis in a short time frame (Alavi et al., 2007; Davies et al., 2007; Nguyen et al., 2011; Sarzi et al., 2012). Zebrafish models have displayed developmental delays in eyes and heads, short length, and body axis abnormalities (Eijkenboom et al., 2019; Rahn et al., 2013) but have not been used to investigate optic nerve degeneration.
In Caenorhabditis elegans, most studies focused on muscle cells using mutants of eat-3, an OPA1 homolog (Kanazawa et al., 2008; Rolland et al., 2009). However, the mitochondria in the posterior lateral microtubule neurons did not show any difference in size from its wild-type (WT) counterparts (Byrne et al., 2019). Thus, LOF of eat-3 may have a limited impact on phenotypic outcomes in the nervous system. Nevertheless, a DOA model in which hOPA1K301A is expressed in GABAergic motor neurons showed a decreased number of mitochondria in those cells (Zaninello et al., 2020). In a fly model, the dOPA1 somatic clone in the eye exhibited a rough-eye phenotype, eye structural abnormalities, and increased MitoSOX fluorescence, which was partially rescued by vitamin E or SOD1 expression (Yarosh et al., 2008). Heterozygous dOPA1 mutants showed shortened lifespan, elevated ROS levels, and irregular muscle tissue mitochondrial structures (Tang et al., 2009). Moreover, the electroretinogram pattern showed age-dependent decreases in the on-transient response, heart rate, negative geotaxis response, and increased heart arrhythmia due to heat shock stress (Shahrestani et al., 2009). However, except for mice, the evidence of optic nerve axonal abnormalities is limited in model organisms such as zebrafish, worms, and flies.
The structure of the Drosophila visual system is similar to that of mammals (Sanes and Zipursky, 2010), with conserved mechanisms of synaptogenesis and neural circuit formation (Sanes and Zipursky, 2020). Drosophila photoreceptors type R7/8 extend their retinal axons from the retina directly into the brain, forming synapses in the second optic ganglion medulla via the chiasma (Figure 1A), having potential application as a model for mammalian photoreceptors and RGCs. In this study, we defined the retinal axons of Drosophila as analogous to the human optic nerve. Based on this, we aimed to develop an experimental model for observing and quantifying degeneration in the Drosophila retinal axon (Nitta et al., 2023; Richard et al., 2022).
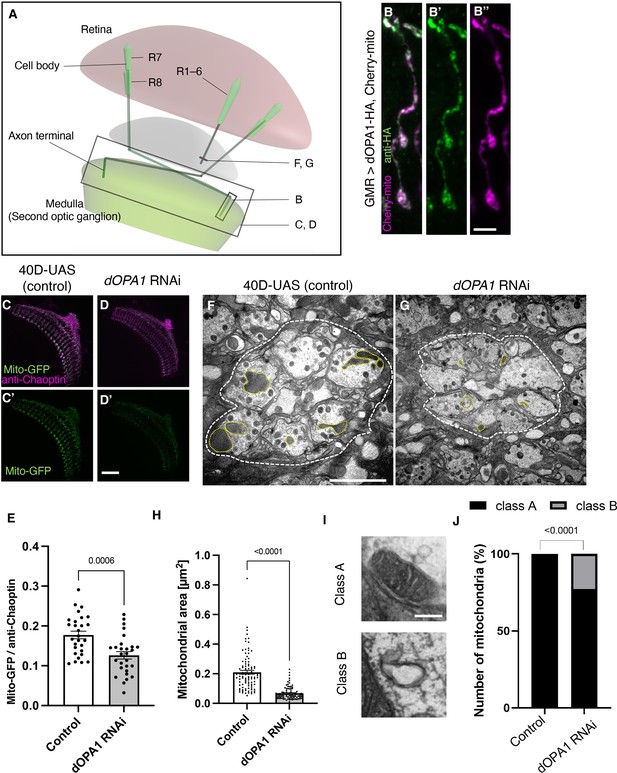
Effect of dOPA1 knockdown on mitochondrial density and size in retinal axons.
(A) Schematic illustration of the Drosophila visual system from a dorsal view, highlighting the arrangement of photoreceptor neurons. The rectangular area indicated in (B) represents a single R7/8 photoreceptor axon, as illustrated in the figure for (B). The rectangular areas highlighted in (C) and (D) encompass the entirety of R7/8 photoreceptor axons, as shown in the figures for (C) and (D). The lines marked in (F) and (G) denote cross-sections of R1–6 photoreceptor axons, as depicted in the figures for (F) and (G). (B) Visualization of mitochondria and dOPA1 in a set of R7/8 axons. Mitochondria were visualized using the expression of mCherry-mito driven by GMR-Gal4 (magenta) while HA-tagged dOPA1 was immunostained using the anti-HA antibody (green). Scale bar: 3 µm. (C, D) Representations of mitochondria visualized using the expression of Mito-GFP driven by GMR-Gal4 (green), 1 day after eclosion. Retinal axons were labeled using the anti-Chaoptin antibody (magenta). Scale bar: 30 µm. (E) Quantification of Mito-GFP intensity. Mito-GFP levels were calculated by dividing the Mito-GFP fluorescence intensity by the anti-Chaoptin signal intensity. Control (n=28 optic lobes) and dOPA1 RNAi (n=28 optic lobes). The data are presented as mean ± SEM. (F, G) Electron micrographs of cross-sections of the R1–6 retinal axons in the lamina of the Control (F) and dOPA1 knockdown (G) on the day of eclosion. Yellow circles and white dotted lines indicate mitochondria and a lamina column, respectively. Scale bar: 2 µm. (H) Quantification of the mitochondrial area. Control (n=96 mitochondria) and dOPA1 RNAi (n=92 mitochondria). Data are expressed as mean ± SEM. (I) Representative EM images showing mitochondria with a densely packed matrix structure (classified as Class A) and collapsed mitochondria (classified as Class B). Scale bar: 500 nm. (J) Quantification of the mitochondria classified into Class A and Class B. Control (n=96 mitochondria) and dOPA1 RNAi (n=92 mitochondria). See the Supplementary file 1 for the genotypes of the Drosophila used in the experiments.
In this study, a dOPA1 LOF could mimic the human DOA, in which mitochondrial fragmentation, increased ROS levels, and neurodegeneration occur in retinal axons. The axonal degeneration induced by the dOPA1 mutant was rescued by the expression of human OPA1 (hOPA1). This demonstrated that the function of hOPA1 is the same as in the fly. Previously reported mutations failed to rescue the dOPA1 deficiency phenotype. To distinguish between the effect of LOF and DN mutations, we expressed the hOPA1 gene of both WT and mutation in a genetic background in which dOPA1 was deleted in retinal axons. The DOA plus mutations D438V and R445H inhibited the rescue by the WT of hOPA1. Taken together, we generated a new Drosophila DOA model which served to isolate the LOF or DN effect of hOPA1 mutations.
Results
dOPA1 depletion caused mitochondrial fragmentation in axon terminals
The OPA1 protein is located in the inner mitochondrial membrane. To confirm whether dOPA1 is also localized in the mitochondria of Drosophila retinal axons, we used a Gal4/UAS system (Brand and Perrimon, 1993) and expressed HA-tagged dOPA1 proteins using the eye-specific GMR-Gal4 driver (Newsome et al., 2000a; Newsome et al., 2000b). The outer membrane of the mitochondria was visualized with mCherry-mito, which tagged the transmembrane domain of Miro with mCherry (Vagnoni and Bullock, 2016). Confocal microscopy revealed that the HA signals colocalized with mCherry-mito in the axon terminals of photoreceptor cells (Figure 1B). This indicated that dOPA1 colocalizes with mitochondria in retinal axons.
To examine the impact of dOPA1 on mitochondrial structure and density, we analyzed mitochondria in dOPA1 RNAi flies. The intensity of the mitochondrial signal was significantly reduced in the retinal axons of these flies (Figure 1C and D; quantification in Figure 1E). Previously, impaired mitochondrial transport was reported in the LOF of marf (MFN 1/2 homolog), which is necessary for the fusion of the outer membrane of mitochondria (Sandoval et al., 2014). Thus, we investigated if the LOF of dOPA1 may cause transport defects, which may be responsible for the reduced mitochondrial density in retinal axons in dOPA1 RNAi flies. To this end, we observed the photoreceptor neurons after puparium formation at 24 hr, which allowed us to simultaneously visualize the cell bodies and axon terminals of the photoreceptors (Figure 1—figure supplement 1). Both dOPA1 knockdown and control flies showed mitochondrial signals in the axon terminals (Figure 1—figure supplement 1). However, no mitochondrial signals were observed in the axon terminals of flies with a knockdown of milton, which is thought to act as an adaptor between kinesin to mitochondria, promoting anterograde transport (Figure 1—figure supplement 1). These findings suggest that trafficking defects are not the main cause of the mitochondrial mislocalization observed in the retinal axons of the dOPA1 knockdown.
To further analyze the mitochondrial morphology of retinal axons in the LOF of dOPA1, we conducted an electron microscopy analysis (Figure 1F and G). Although Drosophila has eight types of photoreceptors, the axons of R1–6, which project to the lamina of the first optic lobe, can be identified without markers; thus, we chose them for this experiment. The dOPA1 knockdown led to a significant decrease in mitochondrial size in R1–6 axons (Figure 1F–H). In addition, there was significant heterogeneity in healthy mitochondria, ranging from dense matrix (Class A) to swollen mitochondria with a hypodense matrix (Class B) (Figure 1I). Knocking down dOpa1 in R1–6 axon terminals resulted in severely abnormal mitochondrial ultrastructure (22.8%; Figure 1J). These results suggest that dOPA1 is involved in maintaining proper mitochondrial morphology by promoting mitochondrial fusion in retinal axons.
To verify whether ROS is increased in the retinal axons in dOPA1 knockdown photoreceptors, we measured the ROS levels in the knockdown retinal axons using MitoSOX, a mitochondrial superoxide indicator (Yarosh et al., 2008). The results showed significantly elevated ROS levels in dOPA1 knockdown retinal axons compared to control (Figure 2A and B; quantification in Figure 2C).
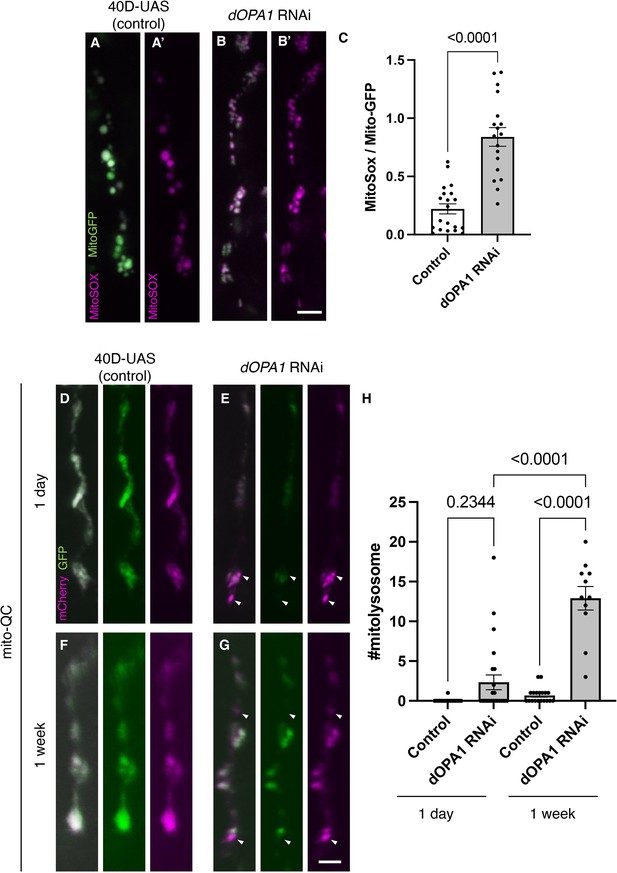
Elevated reactive oxygen species (ROS) and mitophagy activity levels in the dOPA1 knockdown.
(A, B) Mitochondrial ROS levels in a set of the R7/8 retinal axon visualized using Mito-GFP (green) driven by GMR-Gal4 and MitoSOX (magenta) to specifically detect superoxide, an indicator of oxidative stress. Representative images of a retinal axon in the Control (A, A') and dOPA1 knockdown (B, B'). (C) Quantification of ROS levels for each genotype. ROS levels were determined by dividing the fluorescence intensity of MitoSOX by that of Mito-GFP, highlighting the significant increase in ROS levels in dOPA1 knockdown flies compared to controls, suggesting enhanced mitochondrial stress and dysfunction upon dOPA1 depletion. Control (n=20 optic lobes) and dOPA1 RNAi (n=18 optic lobes). Scale bar: 3 µm. Data are presented as mean ± SEM. (D, E) Mitophagy activity in a set of the R7/8 retinal axon visualized by a genetic marker, mitoQC, driven by GMR-Gal4. Representative images of a retinal axon in the Control 1 day after eclosion (D), dOPA1 knockdown 1 day after eclosion (E), the Control 1 week after eclosion (F), dOPA1 knockdown 1 week after eclosion (G). The arrowheads indicate a mitolysosome that is positive for mCherry and negative for GFP. (H) Quantification of the mitophagy activity each genotype. The number of the mitolysosomes were counted in each genotype. The significant increase in the number of the mitolysosomes in dOPA1 knockdown flies compared to controls after 1 week, suggesting enhanced mitophagy upon dOPA1 depletion. Control 1d (n=18 optic lobes), Control 1 week (n=19 optic lobe), dOPA1 RNAi 1d (n=24 optic lobes), and dOPA1 RNAi 1 week (n=11 optic lobes). Scale bar: 3 µm. Data are presented as mean ± SEM. See the Supplementary file 1 for the genotypes of the Drosophila used in the experiments.
Given that an increase in mitophagy activity has been reported in mouse RGCs and nematode ADOA models (Zaninello et al., 2022; Zaninello et al., 2020), the mitoQC marker, an established indicator of mitophagy activity, was expressed in the photoreceptors of Drosophila. The mitoQC reporter consists of a tandem mCherry-GFP tag that localizes to the outer membrane of mitochondria (Lee et al., 2018). This construct allows the measurement of mitophagy by detecting an increase in the red-only mCherry signal when the GFP is degraded after mitochondria are transported to lysosomes. Post dOPA1 knockdown, we observed a significant elevation in mCherry-positive and GFP-negative puncta signals at 1 week, demonstrating an activation of mitophagy as a consequence of dOPA1 knockdown (Figure 2D–H).
The dOPA1 LOF leads to progressive distal degeneration of Drosophila photoreceptors
We tested whether knocking down dOPA1 causes optic nerve degeneration in Drosophila. To this end, we performed an RNAi experiment using the GMR-Gal4 driver and evaluated the number of retinal axons projecting to the second optic ganglion medulla (Figure 3A). To compare the number of retinal axon terminals – R7 axons – between genotypes, we used a previously developed automated method, MeDUsA (method for the quantification of degeneration using fly axons) (Nitta et al., 2023). We have assessed the extent of their reduction from the total axonal terminal count, thereby determining the degree of axonal terminal degeneration (Nitta et al., 2023; Richard et al., 2022). Our results showed that the dOPA1 knockdown caused axonal degeneration 1 day after eclosion, further decreasing 1 week later (Figure 3B–E, quantification in Figure 3F). We obtained similar results with an independent alternative RNAi line (Figure 3—figure supplement 1). To determine whether the degeneration was limited to the axons, we counted the number of rhabdomeres in the cell bodies. Each compound eye comprises 700–800 ommatidia, with each ommatidium containing eight types of photoreceptors, designated as R1–R8. The distal region of an ommatidium reveals the R1–R7 photoreceptors with a stereotypical arrangement of rhabdomeres (arrowheads in Figure 3G–J). Since it is easy to evaluate retinal degeneration by counting the number of rhabdomeres, a decrease in their number indicates degeneration of the photoreceptor cell body. The dOPA1 knockdown caused a significant decrease in the number of rhabdomeres per ommatidium in 1-week-old adults (Figure 3G–J, quantification in Figure 3K). Interestingly, although the retinal axons had already decreased on the day of eclosion, the ommatidia remained intact (Figure 3F and K). Note that the ommatidia in R7 remained intact after 1 week (Figure 3J). Our results indicate that the dOPA1 knockdown causes degeneration in both axonal terminals and cell bodies, with the neuronal degeneration starting from the axons and continuing in the cell bodies. A previous report indicated that the compound eyes of homozygous mutations of dOPA1 displayed a glossy eye phenotype (Yarosh et al., 2008). Upon knocking down dOPA1 using the GMR-Gal4 driver, we also observed a glossy eye-like rough-eye phenotype in the compound eyes (Figure 3—figure supplement 2). The GMR-Gal4 driver does not exclusively target Gal4 expression to photoreceptor cells. Consequently, the observed retinal axonal degeneration could potentially be secondary to abnormalities in support cells external to the photoreceptors.
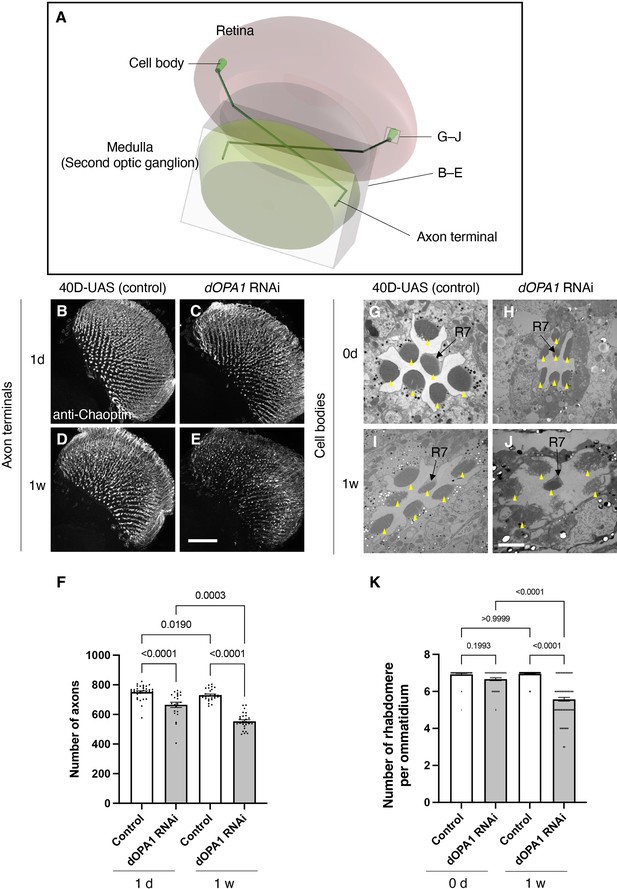
Effect of dOPA1 knockdown on photoreceptor neurodegeneration in Drosophila.
Schematic of the visual system in Drosophila. A set of R7/8 photoreceptors project their axons to the second optic ganglion medulla. The rectangular prism indicated in B–E represents the area of the whole medulla, as shown in the figure for (B–E). The square marked in G–J denote cross-sections of the cell bodies of the photoreceptors, as depicted in the figures for (G–J). (B–E) All R7 and R8 axon terminals project to the medulla. dOPA1 in the photoreceptor was knocked down by GMR-Gal4. 40D-UAS was used as a Control to match the number of UAS sequences recognized by Gal4. One day after eclosion in Control (B) and dOPA1 knockdown (C), and 1 week after in Control (D) and dOPA1 knockdown (E). The retinal axons were stained with anti-Chaoptin, a photoreceptor-specific antibody. Scale bar = 50 μm. (F) Number of axons at each time point in R7 neurons for each situation quantified by MeDUsA (method for the quantification of degeneration using fly axons). At 1 day of Control (n=34 optic lobes) and dOPA1 RNAi (n=23 optic lobes), and at 1 week of Control (n=28 optic lobes) and dOPA1 RNAi (n=26 optic lobes). Data are expressed as mean ± SEM. (G–J) Electron micrographs of cross-sections of the photoreceptor cell bodies in the ommatidia of the retina. Rhabdomeres are shown as yellow arrowheads. The day of eclosion in Control (G) and dOPA1 knockdown (H), and 1-week-old adults of Control (I) and dOPA1 knockdown (J). Scale bar = 2 μm. (K) Quantification of the number of rhabdomeres for each genotype and time point. At 0 day in Control (n=50 ommatidia) and dOPA1 RNAi (n=127 ommatidia), and at 1 week in Control (n=50 ommatidia) and dOPA1 RNAi (n=117 ommatidia). Data are expressed as mean ± SEM. See the Supplementary file 1 for the genotypes of the Drosophila used in the experiments.
To test whether our RNAi results reflect dOPA1 downregulation, we performed mutant analysis of the dOPA1 gene in retinal axons. For this, we used the dOPA1s3475 hypomorphic mutant allele, which has a P-element insertion in exon 2 (Yarosh et al., 2008). Somatic mosaic clones were generated in the retinal axons using the FLP/FRT system (Golic and Lindquist, 1989) because homozygous mutant alleles are embryonic lethal (Sandoval et al., 2014; Yarosh et al., 2008). Note that the mutant clone analysis was conducted in a context where mutant and heterozygous cells coexist as a mosaic, and it was not possible to distinguish between them. Using MeDUsA, we found a significantly lower number of dOPA1 mutant retinal axons than in controls 1 day after eclosion (Figure 4A and B, quantification in Figure 4D). To determine if dOPA1 is responsible for axon neurodegeneration, we quantify the number of the axons in the dOPA1 eye clone fly with the expression of dOPA1 at 1 day after eclosion and found that dOPA1 expression partially rescued the axonal degeneration (Figure 4C, quantification in Figure 4D). Our results indicate that dOPA1 is necessary for maintaining the number of retinal axons in the Drosophila visual system. In conclusion, the neurodegeneration observed in the LOF of dOPA1 is due to the progressive loss of retinal axons, as well as the mammal optic nerve.
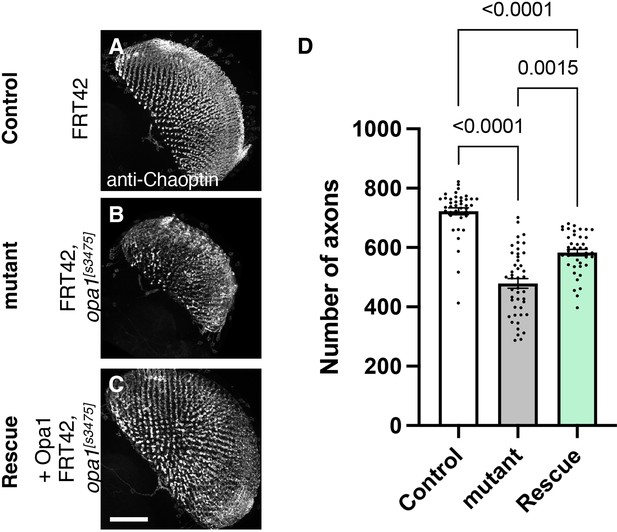
Role of dOPA1 in the retinal axon according to mutant analysis.
(A–C) Representations of retinal axons labeled with an anti-Chaoptin antibody (gray), 1 day after eclosion. The images show Control (A), dOPA1s3475 somatic mosaic flies (B), and dOPA1s3475 somatic mosaic flies expressing the eye-specific full-length dOPA1 (C). Scale bar: 50 µm. (D) Quantification of the number of axons for each genotype. Control (n=41 optic lobes), mutant (n=45 optic lobes), and rescue (n=41 optic lobes). The data are presented as mean ± SEM. See the Supplementary file 1 for the genotypes of the Drosophila used in the experiments.
Investigating disease mutations in OPA1 using a fly DOA model to confirm their pathological significance
Our observations in dOPA1 LOF flies confirmed mitochondrial fragmentation, increased ROS levels, and degeneration of retinal axons, as reported in mammals. These data indicated that the function of OPA1 may be conserved across Drosophila and humans. Thus, we tested whether hOPA1 could effectively replace dOPA1 in flies by generating a transgenic organism to express hOPA1 in Drosophila. Following the UAS sequence, that the yeast-derived transcription factor Gal4 binds, the construct included an HA tag, followed by the hOPA1 gene, and a myc tag (Figure 5A). This construct was expressed in Drosophila and protein expression was confirmed by western blotting. The HA tag, attached to the N-terminus, was immunoblotted resulting in a band of the expected full-length size (Figure 5B). For the myc tag at the C-terminus, a strong band and two weak bands were observed at the upper and lower positions (Figure 5B). Comparison of the expression levels across the variants revealed no significant differences in protein expression (Figure 5C). We also employed a human anti-OPA1 antibody to verify the expression of hOPA1 in vivo in Drosophila, detecting both the long and short forms of OPA1, band 2 and band 4, respectively (Figure 5D).
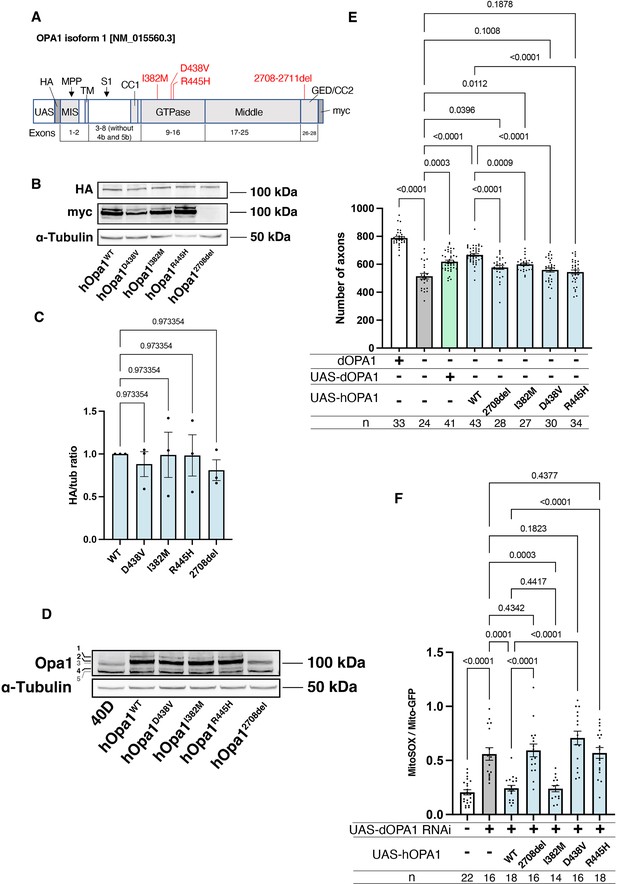
Verification of the pathological significance of disease mutations in hOPA1.
(A) Schematic illustration of the hOPA1 gene construct with HA and myc tags in the UAS-based vector. hOPA1 includes a mitochondrial import sequence (MIS) cleaved by mitochondrial processing peptidase (MPP), a transmembrane region (TM), a coiled-coil region (CC1), a GTPase domain, a Middle domain, and a GTPase effector domain (GED) containing a coiled-coil region (CC2). The sites of variants (I382M, D438V, R445H, and a deletion from 2708 to 2711) are shown in red. S1 is the site cleaved by OMA1. (B) Western blot analysis to confirm the expression of hOPA1 variants. hOPA1_WT, hOPA1_D438V, hOPA1_I382M, hOPA1_R445H, and hOPA1_2708del were expressed in whole Drosophila bodies and detected using anti-HA and anti-myc antibodies. α-Tubulin was used as a loading Control. (C) Quantification of the expression levels of the OPA1 protein for each variant. hOPA1_WT (n=3), D438V (n=3), I382M (n=3), R445H (n=3), and 2708del (n=3). The data are presented as mean ± SEM. (D) Detection of hOPA1 expression in vivo in Drosophila using anti-OPA1. Band 1 represents the full-length OPA1, which includes the MIS, while Band 2 corresponds to the long-form OPA1 (L-OPA1), cleaved at the MPP. Bands 3 and 5 potentially detect the endogenous dOPA1. Band 4 is thought to represent the short-form OPA1 (S-OPA1). (E) Rescue experiments were conducted to assess the expression of each hOPA1 variant, including dOPA1, in the retina axons of dOPA1 mutant somatic clones. The sample size is indicated (n). Data are presented as mean ± SEM. (F) Quantification of mitochondrial reactive oxygen species (ROS) levels upon expression of hOPA1 variants in the context of dOPA1 knockdown. Control (n=22 optic lobes), dOPA1 RNAi (n=16 optic lobes), hOPA1_WT with dOPA1 RNAi (n=18 optic lobes), hOPA1_2708-2711del with dOPA1 RNAi (n=16 optic lobes), hOPA1_I382M with dOPA1 RNAi (n=14 optic lobes), hOPA1_D438V with dOPA1 RNAi (n=16 optic lobes), and hOPA1_R445H with dOPA1 RNAi (n=18 optic lobes). Data are presented as mean ± SEM. See the Supplementary file 1 for the genotypes of the Drosophila used in the experiments.
Using this UAS-hOPA1, we performed rescue experiments. hOPA1 expression in the retinal axons of the dOPA1 mutant, which generated somatic cell clones, partially rescued the number of axons (Figure 5E). These results suggest that hOPA1 and dOPA1 are interchangeable. We also generated and expressed the hOPA1 mutation 2708-2711del, which is known to cause DOA, the I382M mutation, located in the GTPase domain and associated with DOA, as well as the D438V and R445H mutations, also located in the GTPase domain and associated with DOA plus. The expression of these mutations in retinal axons failed to rescue the dOPA1 deficiency to the same extent as the WT hOPA1 (Figure 5E). Importantly, unlike the D438V and R445H mutations, the 2708-2711del and I382M mutations could be weakly rescued. This suggests that these mutations result in LOF, but may retain some residual activity. These findings are also in line with the observed severity of the associated diseases, DOA and DOA plus. The D438V and R445H mutations were not significantly rescued, unlike the 2708-2711del and I382M mutations. Our methodology distinctively facilitates the quantitative evaluation of LOF severity by comparing the rescue capabilities of various mutations. Notably, the 2708-2711del and I382M mutations demonstrated only partial rescue, indicative of a hypomorphic effect with residual activity. In contrast, the D438V and R445H mutations failed to show significant rescue, suggesting a more profound LOF. The correlation between the partial rescue by the 2708-2711del and I382M mutations and their classification as hypomorphic is significant. Moreover, the observed differences in rescue efficacy correspond to the clinical severities associated with these mutations, namely in DOA and DOA plus disorders. Thus, our results substantiate the model’s ability to quantitatively discriminate among mutations based on their impact on protein functionality, providing an insightful measure of LOF magnitude.
Furthermore, we assessed the potential for rescuing ROS signals. Similar to its effect on axonal degeneration, WT hOPA1 effectively mitigated the phenotype, whereas the 2708-2711del, D438V, and R445H mutants did not (Figure 5F). Importantly, the I382M variant also reduced ROS levels comparably to the WT. These findings demonstrate that both axonal degeneration and the increase in ROS caused by dOPA1 downregulation can be effectively counteracted by hOPA1. Although I382M retains partial functionality, it acts as a relatively weak hypomorph in this experimental setup.
We also conducted western blot analyses using anti-COXII and anti-Atg8a antibodies to assess changes in mitochondrial quantity and autophagy activity following the knockdown of dOPA1. Mitochondrial protein levels, indicated by COXII quantification, were evaluated to verify mitochondrial content, and the ratio of Atg8a-1 to Atg8a-2 was used to measure autophagy activation. For these experiments, Tub-Gal4 was employed to systemically knock down dOPA1. Considering the lethality of a whole-body dOPA1 knockdown, Tub-Gal80TS was utilized to repress the knockdown until eclosion by maintaining the flies at 20°C. After eclosion, we increased the temperature to 29°C for 2 weeks to induce the knockdown or expression of hOPA1 variants. The results revealed no significant differences across the genotypes tested (Figure 5—figure supplement 1).
Distinction between LOF and DN mutations in hOPA1 linked to DOA or DOA plus
Currently, the hOPA1 mutations that contribute to DOA plus have been primarily located in the GTPase domain of hOPA1. The role of hOPA1 as a GTPase is facilitated by its ability to form a polymer via the GED domain and Middle domain (Li et al., 2019). This led to the hypothesis that mutations in the GTPase domain can interact with WT hOPA1 but cannot activate the GTPase activity to show a DN effect. However, it has been challenging to clarify whether these mutations are DN or LOF, as there is a significant number of LOF mutations in the GTPase domain. Given that dOPA1 can be substituted with hOPA1, we suspected that a DN effect could also be achieved by expressing the D438V or R445H mutations in the WT background. However, the axons did not degenerate despite expression and monitoring for 2 weeks in adult flies (Figure 6A). The results presented in Figure 5C indicate that there are no significant differences in the expression levels among the variants, suggesting that variations in expression levels do not influence the outcomes. The amino acid sequences of hOPA1 and dOPA1 are highly conserved (72% identity), particularly in the GTPase domain. The Middle and GED domains are also relatively well preserved, with 54% and 64% agreement, respectively (Figure 6—figure supplement 1). These results imply that, although dOPA1 and hOPA1 are functionally interchangeable, they may not interact with each other. Consequently, we expressed hOPA1 in the photoreceptor depleted of dOPA1 by somatic clone; under these conditions, we further expressed hOPA1 WT, 2708-2711del, and mutations in the GTPase domains, including R445H, I382M, and D438V. This allowed hOPA1 to interact with itself and to verify the DN effect. As a result, the expression of the WT, 2708del, and I382M did not result in any changes when rescued by hOPA1 substitution (Figure 6B). However, the rescue was significantly suppressed for D438V and R445H, which are known to cause DN; thus, the DN effect could be replicated (Figure 6B). In conclusion, we established an experimental model that can separate LOF and DN, the pathological significance of hOPA1 mutations. We investigated the impacts of DN mutations on mitochondrial oxidation levels, mitochondrial quantity, and autophagy activation levels; however, none of these parameters showed statistical significance (Figure 6—figure supplement 2).
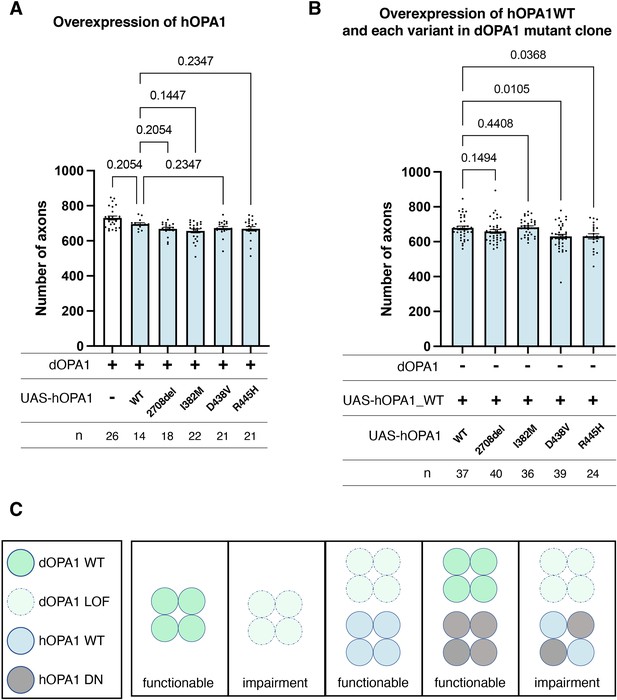
Loss-of-function (LOF) or dominant-negative (DN) effects of disease mutations in hOPA1.
(A) Impact of each human OPA1 variant on the axon number in the optic nerve of Drosophila as quantified using MeDUsA (method for the quantification of degeneration using fly axons). (B) Expression of both hOPA1 wild-type (WT) and its respective variants was analyzed in photoreceptors lacking dOPA1, and the number of axons was quantified using the MeDUsA. The sample size is indicated (n). Data are expressed as mean ± SEM. (C) Schematic representation of interspecies differences in OPA1 interactions and the interchangeability of OPA1 between human and fly. See the Supplementary file 1 for the genotypes of the Drosophila used in the experiments.
Discussion
In this study, we found that LOF of dOPA1 in Drosophila can imitate human DOA, in which the optic nerve degenerates. We were successful in reversing this degeneration by expressing hOPA1 (Figure 6C). However, we could not rescue any previously reported mutations known to cause either DOA or DOA plus except for I382M. In the context of rescuing the retinal axons of the dOPA1 mutant by expressing the WT of hOPA1, it was observed that only the DOA plus mutations suppressed the rescue (Figure 6C). This allowed us to distinguish between LOF and DN mutations in hOPA1. The fly model developed in this study will allow investigating new hOPA1 mutations for DOA or DOA plus. We have previously utilized MeDUsA to quantify axonal degeneration, applying this methodology extensively to various neurological disorders. The robust adaptability of this experimental system is demonstrated by its application in exploring a wide spectrum of genetic mutations associated with neurological conditions, highlighting its broad utility in neurogenetic research. We identified a novel de novo variant in Spliceosome Associated Factor 1, Recruiter of U4/U6.U5 Tri-SnRNP (SART1). The patient, born at 37 weeks with a birth weight of 2934 g, exhibited significant developmental delays, including an inability to support head movement at 7 months, reliance on tube feeding, unresponsiveness to visual stimuli, and development of infantile spasms with hypsarrhythmia, as evidenced by EEG findings. Profound hearing loss and brain atrophy were confirmed through MRI imaging. To assess the functional impact of this novel human gene variant, we engineered transgenic Drosophila lines expressing both WT and mutant SART1 under the control of a UAS promoter. Our MeDUsA analysis suggested that the variant may confer a gain-of-toxic-function (Nitta et al., 2023). Moreover, we identified heterozygous LOF mutations in DHX9 as potentially causative for a newly characterized neurodevelopmental disorder. We further investigated the pathogenic potential of a novel heterozygous de novo missense mutation in DHX9 in a patient presenting with short stature, intellectual disability, and myocardial compaction. Our findings indicated a LOF in the G414R and R1052Q variants of DHX9 (Yamada et al., 2023). This experimental framework has been instrumental in elucidating the impact of gene mutations, enhancing our ability to diagnose how novel variants influence gene function. Our research established that dOPA1 knockdown precipitates axonal degeneration and elevates ROS signals in retinal axons. Expression of human OPA1 within this context effectively mitigated both phenomena; it partially reversed axonal degeneration and nearly completely normalized ROS levels. These results imply that factors other than increased ROS may drive the axonal degeneration observed post knockdown. Furthermore, while differences between the impacts of DN mutations and LOF mutations were evident in axonal degeneration, they were less apparent when using ROS as a biomarker. The extensive use of transgenes in our experiments might have mitigated the knockdown effects. In a systemic dOPA1 knockdown, assessments of mitochondrial quantity and autophagy activity revealed no significant changes, suggesting that the cellular consequences of reduced OPA1 expression might vary across different cell types.
In our study, expressing dOPA1 in the retinal axons of dOPA1 mutants resulted in significant rescue, but it did not return to control levels. There are three possible explanations for this result. The first concerns gene expression levels. The Gal4-line used for the rescue experiments may not replicate the expression levels or timing of endogenous dOPA1. Considering that the optimal functionality of dOPA1 may be contingent upon specific gene expression levels, attaining a WT-like state necessitates the precise regulation of these expression levels. The second is a non-autonomous issue. Although dOPA1 gene expression was induced in the retinal axons for the rescue experiments, many retinal axons were homozygous mutants, while other cell types were heterozygous for the dOPA1 mutation. If there is a non-autonomous effect of dOPA1 in cells other than retinal axons, it might not be possible to restore the WT-like state fully. The third potential issue is that only one isoform of dOPA1 was expressed. In mouse OPA1, to completely restore mitochondrial network shape, an appropriate balance of at least two different isoforms, long-form OPA1 (L-OPA1) and short-form OPA1 (s-OPA1), is required (Del Dotto et al., 2017). This requirement implies that multiple isoforms of dOPA1 are essential for the dynamic activities of mitochondria.
Our established fly model is the first simple organism to allow observation of degeneration of the retinal axons. The mitochondria in the axons showed fragmentation of mitochondria. Former studies have observed mitochondrial fragmentation in S2 cells (McQuibban et al., 2006), muscle tissue (Deng et al., 2008), segmental nerves (Trevisan et al., 2018), and ommatidia (Yarosh et al., 2008) due to the LOF of dOPA1. Our study presents compelling evidence that dOPA1 knockdown instigates neuronal degeneration, characterized by a sequential deterioration at the axonal terminals and extending to the cell bodies. This degenerative pattern, commencing from the distal axons and progressing proximally toward the cell soma, aligns with the paradigm of ‘dying-back’ neuropathy, a phenomenon extensively documented in various neurodegenerative disorders (Wang et al., 2012). Previously, the function of OPA1 in mice (mOPA1) was substituted by hOPA1 (Del Dotto et al., 2017; Sarzi et al., 2018). Here, we demonstrated that the function of dOPA1 can also be substituted by hOPA1. Thus, the function of OPA1 is conserved across a wide range of species but our fly model has the advantage of allowing rapid observation of phenotypes. In the mouse model of DOA with the heterozygous deletion of the mOPA1 gene at positions 329–355 (Alavi et al., 2007) or delTTAG mutant (Sarzi et al., 2012), a decrease in the number of RGC was observed after 17 months or 16 months of age, respectively. To save time, using a fly model for quick analysis and direct observation of retinal axons would be useful. Previous models using nematodes, flies, and zebrafish did not observe axonal degeneration directly, but our new model has made this possible. As a result, our model has the potential to be used for screening for modifiers of unclear molecular pathologies and drug screening.
Our model could also demonstrate the pathological significance of hOPA1 mutations and be used for genetic diagnosis to differentiate between LOF and DN mutations. To elucidate the pathophysiological implications of mutations in the OPA1 gene, we engineered and expressed several human OPA1 variants, including the 2708-2711del mutation, associated with DOA, and the I382M mutation, located in the GTPase domain and linked to DOA. We also investigated the D438V and R445H mutations in the GTPase domain and correlated with the more severe DOA plus phenotype. The 2708-2711del mutation exhibited limited detectability via HA-tag probing. Still, it was undetectable with a myc tag, likely due to a frameshift event leading to the mutation’s characteristic truncated protein product, as delineated in prior studies (Zanna et al., 2008). Contrastingly, the I382M, D438V, and R445H mutations demonstrated expression levels comparable to the WT hOPA1.
However, the expression of these mutants in retinal axons did not restore the dOPA1 deficiency to the same extent as the WT hOPA1, as evidenced in Figure 5E. This finding indicates a functional impairment imparted by these mutations, aligning with established understanding (Zanna et al., 2008). Notably, while the 2708-2711del and I382M mutations exhibited limited functional rescue, the D438V and R445H mutations did not show significant rescue activity. This differential rescue efficiency suggests that the former mutations, particularly the I382M, categorized as a hypomorph (Del Dotto et al., 2018), may retain partial functional capacity, indicative of a LOF effect but with residual activity. The I382M missense mutation within the GTPase domain of OPA1 has been described as a mild hypomorph or a disease modifier. Intriguingly, this mutation alone does not induce significant clinical outcomes, as evidenced by multiple studies (Bonifert et al., 2014; Bonneau et al., 2014; Carelli et al., 2015; Schaaf et al., 2011). A significant reduction in protein levels has been observed in fibroblasts originating from patients harboring the I382M mutation. However, mitochondrial volume remains unaffected, and the fusion activity of mitochondria is only minimally influenced (Del Dotto et al., 2018; Kane et al., 2017). This observation is consistent with findings reported by Chao de la Barca et al., 2020, where a targeted metabolomics approach classified I382M as a mild hypomorph. In our current study, the I382M mutation preserves more OPA1 function compared to DN mutations, as depicted in Figure 5E and F. Considering the results from our Drosophila model and previous research, we hypothesize that the I382M mutation may constitute a mild hypomorphic variant. This might explain its failure to manifest a phenotype on its own, yet its contribution to increased severity when it occurs in compound heterozygosity.
Our results for LOF or DN segmentation were consistent with previously reported yeast models (Del Dotto et al., 2018) and fibroblasts obtained from patients with OPA1 mutations (Kane et al., 2017), and confirmed the conserved function of OPA1 across species. A major advantage of the Drosophila model over yeast or fibroblasts is that we can observe a phenotype that mimics the actual disease state of axonal degeneration in retinal axons and analyze it not only at the molecular level, but also the structural level of axons and mitochondria. The transgenic Drosophila of disease-associated hOpa1 mutations created in this study can also serve to understand the pathophysiology of these mutations.
Advances in genome analysis allowed the identification of many variants and genetic mutations associated with DOA using next-generation sequencing. This could confirm diagnoses and identify new forms of DOA. The widespread use of these sequencers has also created new challenges, such as the growing number of variants of unknown significance (VUS) in publicly available databases such as CliniVar for DOA. These variants are categorized as pathogenic, likely pathogenic, VUS, likely benign, or benign, and the number of VUS is increasing as more variants are registered. The ClinVar database had classified 110 out of 319 Opa1 variants as VUS at the end of 2019. By the end of 2022, this number had increased to 357 out of 840, indicating an increase from 34% to 42% over the last 3 years (Henrie et al., 2018). This trend suggests that the number of VUS in Opa1 may continue to increase in the future. Analyzing VUS requires testing the effects of a mutation in vitro or in vivo; this can be expensive and time-consuming without prior confirmation or high probability of pathological significance. However, our fly model can be used to easily and inexpensively determine the pathological effects of disease-causing gene mutations among the growing number of rare mutations identified in DOA.
In this study, we tested the effect of expressing the D438V and R445H mutations in the dOPA1 background and found that retinal axons did not degenerate. Regarding the interactions among OPA1 proteins, in yeast Mgm1, K854 in the region near the C-terminus is important for aggregation, and it becomes an oligomer to increase the activity of GTPase (Rujiviphat et al., 2009). Although hOPA1 normally has low GTPase activity, its interaction with negatively charged phospholipids such as cardiolipin causes hOPA1 to aggregate, increasing GTP hydrolysis activity (Ban et al., 2010). In the thermophilic fungus Chaetomium thermophilum, Mgm1 forms a dimer and then a tetramer at the C-terminal Stalk domain of Mgm1 (Faelber et al., 2019). Structural analysis also predicted that the Middle and GED domains are necessary for dimer and oligomer formation in hOPA1 (Li et al., 2019). In addition, peptide-binding assays have revealed that the coiled-coil domain, which is part of the GED domain, is required for hOPA1 interactions (Akepati et al., 2008). Comparing the amino acid sequences of hOPA1 and dOPA1, the Middle and GED domains, which are necessary for interactions between OPA1 molecules, exhibit concordance rates of 54.3% and 63.6%, respectively. These percentages are low compared to the 71.8% identity observed in the GTPase domains. These suggest that the OPA1 homologs of each species form oligomers with their unique OPA1. Note that the expression of dOPA1K273A, a presumably GTPase-negative form of dOPA1 (Tsuyama et al., 2017), degenerated retinal axons (Figure 6—figure supplement 3). These results imply that, while dOPA1 and hOPA1 are interchangeable, they may not interact with each other. This could pave the way for the development of a chimeric hOPA1, which would retain its functional properties while avoiding the interaction with endogenous hOPA1. Such chimeric hOPA1 could potentially be used as gene therapy since induced pluripotent stem cells have been generated from a patient with R445H (Sladen et al., 2021).
OPA1 has been implicated in various other diseases as well, including normal tension glaucoma (Powell et al., 2003; Yu-Wai-Man et al., 2010b), multiple sclerosis-like illness (Verny et al., 2008; Yu-Wai-Man et al., 2016), Parkinson’s disease and dementia (Carelli et al., 2015; Lynch et al., 2017), and cardiomyopathy (Spiegel et al., 2016). Although the connection between these diseases and OPA1 is not fully understood, further research on DOA may provide a deeper insight into this relationship. In the future, pathological analysis using the present model could have implications for understanding the mechanisms underlying these diseases.
DOA has also been reported to involve mtDNA depletion (Amati-Bonneau et al., 2008), but our model, while useful for nuclear gene analysis, is limited for mtDNA analysis. The gene content in the Drosophila mtDNA genome is similar as that in vertebrates, but the gene order and distribution on both DNA strands differ. mOPA1 interacts with mtDNA nucleoids through exon 4b binding to mtDNA D-loops, independent of mitochondrial fusion (Yang et al., 2020). However, the homologous region of mOPA1 or hOPA1 to exon 4b is not found in Drosophila, and the D-loop of mtDNA has not been found in cultured cells of flies or in mtDNA of embryos using electron microscopy (Rubenstein et al., 1977). In addition, while the D-loop is involved in mtDNA replication in humans (Fish et al., 2004), the A+T region is the origin of replication in mtDNA replication in Drosophila (Goddard and Wolstenholme, 1978). Thus, there may be differences in the mechanism of mtDNA replication between humans and flies (Garesse and Kaguni, 2005). Therefore, the association of dOPA1 with mtDNA may differ from that in mammals and the regulatory mechanism of mtDNA homeostasis in which dOPA1 is involved requires further investigation.
Another limitation of our model is that the molecular mechanisms underlying the formation of L-OPA1 and S-OPA1, which are well analyzed in mammals (MacVicar and Langer, 2016), have not been elucidated in Drosophila. In hOPA1, S1 or S2 sites are processed by the i-AAA protease OMA1 and Yme1L respectively to produce short form of hOPA1 (S-hOPA1) (Anand et al., 2014). Despite the fact that Yme1L is conserved in Drosophila, it has been involved in the degradation of the dOPA1 protein rather than its cleavage by dYme1L (Liu et al., 2020). Moreover, the cleavage site for i-AAA does not exist in dOPA1 (Olichon et al., 2007). Regarding other proteases, yeast Mgm1 is processed by the rhomboid protease Pcp1 (Esser et al., 2002; Herlan et al., 2003). However, the Rhomboid-7, a Pcp1 ortholog of Drosophila, was not required for dOPA1 processing (Whitworth et al., 2008). Although hOPA1 expression in Drosophila has identified three different sizes of hOPA1 in our western blotting result, it is unclear how they are cleaved. From a size perspective, the upper band was inferred to represent the full-length hOPA1 including the mitochondrial import sequence (MIS). Since the Middle and the lowest bands were not present in HA-tagged samples, it is possible that the bands at the Middle size represent the long-form hOPA1 in which probably MIS was processed. The band detected at the lowest position may represent an S-hOPA1. However, Drosophila does not have OMA1, an i-AAA protease that cleaves the S1 site. Although another i-AAA protease that cleaves the S2 site, YME1L, is conserved, our expressed hOPA1 is isoform 1 and lacks the S2 site (Figure 5A). Therefore, it is not clear how S-hOPA1 is processed in Drosophila. The anti-dOPA1 antiserum detects three bands in the overexpression of a FLAG-tagged dOPA1 construct (Poole et al., 2010), suggesting a molecular mechanism by which dOPA1, like yeast and mammals, is cleaved after translation. However, the proteases involved in the mechanism are not yet clarified. Whether Drosophila’s endogenous mechanisms can be used to study how L-OPA1 and S-OPA1 are involved in DOA remains unclear.
In this study, we established a new model of DOA in Drosophila, in which we discovered the following: (1) We could replicate the human disease of optic nerve degeneration, allowing rapid genetic disease analysis. (2) We could distinguish the LOF and DN effects of hOPA1 by observation of axonal degeneration phenotype but not with ROS signal, autophagy activity, and amount of the mitochondria in this model. These findings can reveal the pathological significance of de novo mutations and are useful in diagnosing DOA or DOA plus. Additionally, while hOPA1 is the major gene involved in DOA, other genes involved in DOA and interacting proteins have not been investigated yet. Our model can be used for pathological modifier screening, exploring modifiers, and contributing to drug development. In the future, we hope to provide insights that can be applied to the fundamental treatment of DOA.
Materials and methods
Fly strains
Request a detailed protocolFlies were maintained at 25°C on standard fly food. For knockdown experiments (Figure 1C–E, F–J, Figure 2A–H, Figure 3B–K, Figure 5F, Figure 1—figure supplement 1, Figure 3—figure supplement 1, Figure 6—figure supplement 2A, and Figure 6—figure supplement 3), flies were kept at 29°C in darkness. Female flies were used in all experiments to ensure consistency in the number of retinal axons. The following fly strains were obtained from the Bloomington Drosophila Stock Center (BDSC): GMR-Gal4 (BDSC 1104), Tub-Gal4 (BDSC 5138), UAS-dicer2(III) (BDSC 24651), UAS-mito-HA-GFP (BDSC 8443), UAS-mCherry-mito (BDSC 66532), UAS-mitoQC (BDSC 91641), UAS-dOPA1 RNAi (BDSC 32358), UAS-Milton RNAi (BDSC 44477), FRT42D, GMR-hid, l(2)cl-R11(1)/CyO; ey-Gal4, UAS-flp (BDSC 5251), ey3.5-flp (BDSC 35542), Tub-Gal80TS (BDSC 7017), and UAS-luciferase RNAi (BDSC 31603). The following fly strains were obtained from the Vienna Drosophila Resource Center (VDRC): The 40D-UAS (VDRC 60101) and UAS-dOPA1 RNAi (VDRC 106290). The following fly strains were obtained from the Kyoto Drosophila Stock Center (Kyoto): FRT19 (Kyoto 101231), FRT42D (Kyoto 101878), and dOPA1s3475 (Kyoto 111438). The UAS-dOPA1-HA and UAS-dOPA1-HAK273A were generously provided by Dr. T Uemura (Kyoto University, Japan). The dOPA1 clone analysis was performed by inducing flippase expression in the eyes using either ey-Gal4 with UAS-flp or ey3.5-flp, followed by recombination at the chromosomal location FRT42D to generate a mosaic of cells homozygous for dOPA1s3475.
Generation of transgenic flies
Request a detailed protocolThe cDNA for WT, I382M, D438V, R445H, and 2708-2711del forms of hOPA1 (NM_015560.3) were generated by Vectorbuilder Inc (Yokohama, Japan). Then, the HA-hOPA1-myc sequence was amplified and inserted into pJFRC81-10XUAS-IVS-Syn21-GFP-p10 (ID36432, Addgene, USA) digested with NotI and XbaI using primers with the N-terminal containing the Kozak and HA sequences (TTACTTCAGGCGGCCGCGGCCAAAATGTACCCATACGATGTTCCAGATTAC) and the C-terminal containing the myc sequence (TTAAAAACGATTCATTCTAGTTACAGATCCTCTTCTGAGATGAGTTTTTGTTCTTTCTCCTGATGAAGAGCTTCAATG). To confirm production of the complete coding sequence, it was bidirectionally sequenced using the Sanger method. These plasmids were injected into embryos and integrated into the attP2 landing site via ΦC31 recombination (WellGenetics, Taiwan).
Immunohistochemistry and imaging
Request a detailed protocolImmunohistochemistry was performed as described previously (Sugie et al., 2017). Flies were anesthetized on a CO2 pad, and specimens of the correct genotype were selected. Specimens were briefly washed in ethanol for a few seconds before being transferred to a Petri dish containing phosphate-buffered saline (PBS). Using forceps, the proboscis was secured, and the right and left eyes were dissected out with another set of forceps. The proboscis and attached trachea were also removed from the brain. The brains were then carefully transferred with forceps into a 1.5 mL tube containing 150 μL of 0.3% PBT (PBS with Triton X-100) at room temperature.
To fix the tissues, 50 μL of 16% formaldehyde was added to each tube, followed by gentle mixing. The samples were incubated in the fixative for 50 min at room temperature. Subsequent washing involved three rinses with 200 μL of 0.3% PBT, using a micropipette for fluid exchange. After the final wash, the PBT was removed, and the samples were incubated in the primary antibody solution overnight (O/N) at 4°C. This was followed by three washes with 0.3% PBT, after which the secondary antibody solution (diluted in 0.3% PBT) was added, and samples were incubated O/N in the dark at 4°C. After another three washes in 0.3% PBT, the samples were prepared for imaging. The following antibodies were used: rat anti-HA (3F10, 1:400; Roche, Switzerland), mouse anti-Chaoptin (24B10, 1:25; Developmental Studies Hybridoma Bank, USA), rat anti-CadN (DN-Ex#8, 1:50; Developmental Studies Hybridoma Bank), anti-rat Alexa Fluor 488 (1:400; Thermo Fisher Scientific, USA), anti-mouse Alexa Fluor 488 (1:400; Thermo Fisher Scientific), anti-mouse Alexa Fluor 568 (1:400; Thermo Fisher Scientific), and anti-rat Alexa Fluor 633 (1:400; Thermo Fisher Scientific). Specimens were mounted using a Vectashield mounting medium (Vector Laboratories, USA). Images were captured using an FV3000 confocal microscope (Olympus, Japan) or a C2 (Nikon, Japan) confocal microscope for Figure 1—figure supplement 1 and processed using the IMARIS software (Oxford Instruments, UK) or Fiji software, an open-source image analysis software (Schindelin et al., 2012).
Electron microscopy
Request a detailed protocolFly heads were fixed O/N with 2.5% glutaraldehyde and 2% paraformaldehyde in 0.1 M sodium cacodylate buffer. Heads were then post-fixed with 1% osmium tetroxide, dehydrated in ethanol, and embedded in Epon. Ultra-thin tangential sections of the laminas (70 nm) were stained with uranyl acetate and lead citrate. Random retinas or lamina sections were imaged at a magnification of ×100k with a VELETA CCD Camera (Olympus) mounted on a JEM 1010 transmission electron microscope (JEOL, Japan). In the R1–6 axons of the lamina, the areas corresponding to mitochondria were quantified using the freehand line tool in NIS Elements software (Nikon). Concurrently, mitochondria were manually categorized based on their structural characteristics: those with a densely packed matrix were classified as Class A, and those with a collapsed structure as Class B.
Quantification of Mito-GFP intensity in retinal axons and retina
Request a detailed protocolTo measure the relative intensity of Mito-GFP in the photoreceptor R7/8 axons of the medulla, the imaging analysis software IMARIS was used. First, the M1–6 layers of the medulla, including the R7/8 axons, were manually covered using the surface function. Then, the surface area was masked, the surface of the left axons was stained with anti-Chaoptin, and the Mito-GFP signal was generated automatically using the surface function. Finally, the voxel number of the Mito-GFP surface was divided by the voxel number of the R axon surface. These quantifications were performed by experimenters blind to the genotype.
Mitochondrial superoxide detection and quantification
Request a detailed protocolThe ROS level was determined using the MitoSOX Red Mitochondrial Superoxide Indicator (Thermo Fisher Scientific). The procedure involved dissecting adult brains in PBS, transferring them to 5 µM MitoSOX solution, and incubating them for 10 min in the dark at room temperature. The brains were then washed with PBS containing 20% Vectashield (Vector Laboratories), followed by sequential washes with PBS containing 40% and 60% Vectashield. The samples were mounted in Vectashield and scanned using an FV3000 confocal microscope (Olympus). To quantify the ROS level in photoreceptor R7/8 axons, the IMARIS software (Bitplane) was used for imaging analysis. The software generated automatic surfaces of the MitoSOX and Mito-GFP signals. Then, the ROS level was calculated by dividing the voxel number of the MitoSOX surface on the Mito-GFP surface by the voxel number of the Mito-GFP surface. These quantifications were performed by experimenters blind to the genotype.
Measurement of mitophagy levels in retinal axons
Request a detailed protocolThe mitophagy levels in the photoreceptor R7/8 axons of the medulla were analyzed using the mitoQC reporter. Mitolysosomes were identified by referencing the red-only mCherry puncta of mitoQC. The number of mitolysomes of each voxel image was manually quantified with IMARIS software (Bitplane). These quantifications were performed by experimenters blind to the genotype.
Western blot analysis of the whole Drosophila body
Request a detailed protocolWe regulated protein expression temporally across the whole body using the Tub-Gal4 and Tub-GAL80TS system. Flies harboring each hOPA1 variant were maintained at a permissive temperature of 20°C, and upon emergence, females were transferred to a restrictive temperature of 29°C for subsequent experiments. The flies were collected in plastic tubes and sonicated (2×30 s) using a Q55 sonicator (Qsonica, Newtown, CT, USA) in lysis buffer (10 mM Tris–HCl, pH 7.5, 150 mM NaCl, 1 mM EDTA, 2% DDM: 10 µL/mg flies) supplemented with 1:1000 (vol/vol) Protease Inhibitor Cocktail Set III (Calbiochem, USA). Rat anti-HRP-conjugated-HA (3F10, 1: 3000; Proteintech, Germany), mouse anti-myc (9B11, 1: 10000; Cell Signaling Technology, USA), rabbit anti-OPA1 (ab42364, 1: 1000; Abcam, UK), rabbit anti-COXII (1: 100; Murari et al., 2020), rabbit anti-Atg8a (ab109364, 1: 8000; Abcam), mouse anti-α-Tubulin (T9026, 1:100,000; Sigma‐Aldrich, USA), goat HRP-conjugated anti-mouse IgG (SA00001-1, 1: 10,000; Proteintech), and goat HRP-conjugated anti-rabbit IgG (7074S, 1: 5,000; Cell Signaling Technology) antibodies were used for western blotting.
Pairwise alignment of hOPA1 and dOPA1
Request a detailed protocolThe amino acid sequences for hOPA1 and dOPA1 were referenced from NM_015560.3 and NM_166040.2, respectively, and aligned using the EMBOSS Needle (Rice et al., 2000). The GTPase, Middle, and GED domains in dOPA1 were identified by cross-referencing the previously described amino acid positions of these domains in hOPA1 (Liesa et al., 2009). The degree of identity between the aligned amino acid sequences was subsequently determined.
Experimental design and statistical analyses
Request a detailed protocolExperimental analyses were performed using Prism 9 (GraphPad Software, USA). For Figures 1E, H, 2C, Figure 3—figure supplement 1B, and Figure 6—figure supplement 3B, data were analyzed using t-tests (and nonparametric tests) and Mann-Whitney tests. For Figure 1J, Fisher’s exact test was used. For Figures 2H—6, Figure 3—figure supplement 2B, Figure 5—figure supplement 1B, Figure 5—figure supplement 1D, Figure 6—figure supplement 2C, and Figure 6—figure supplement 2E, differences among multiple groups were examined using the Kruskal−Wallis test followed by the two-stage linear step-up procedure of Benjamini, Krieger, and Yekutieli tests. The null hypothesis was rejected at a 0.05 level of significance. Sample sizes are indicated in each figure. The number of retinal axons and rhabdomeres, the intensity of Mito-GFP and MitoSOX, the mitochondrial area and the classification, and the corrected p-values are described in the Results section. All data are expressed as the mean ± SEM.
Data availability
The computer code essential for replicating the axonal degeneration quantification of this study is available on GitHub (copy archived at Kawai et al., 2024). This study does not involve any specific datasets. All relevant data generated or analyzed during this study are included in the manuscript itself. No additional data files are applicable for this research.
References
-
Characterization of OPA1 isoforms isolated from mouse tissuesJournal of Neurochemistry 106:372–383.https://doi.org/10.1111/j.1471-4159.2008.05401.x
-
The association of autosomal dominant optic atrophy and moderate deafness may be due to the R445H mutation in the OPA1 geneAmerican Journal of Ophthalmology 136:1170–1171.https://doi.org/10.1016/s0002-9394(03)00665-2
-
The i-AAA protease YME1L and OMA1 cleave OPA1 to balance mitochondrial fusion and fissionThe Journal of Cell Biology 204:919–929.https://doi.org/10.1083/jcb.201308006
-
Disruption of mitochondrial dynamics affects behaviour and lifespan in Caenorhabditis elegansCellular and Molecular Life Sciences 76:1967–1985.https://doi.org/10.1007/s00018-019-03024-5
-
Syndromic parkinsonism and dementia associated with OPA1 missense mutationsAnnals of Neurology 78:21–38.https://doi.org/10.1002/ana.24410
-
Metabolomics hallmarks OPA1 variants correlating with their in vitro phenotype and predicting clinical severityHuman Molecular Genetics 29:1319–1329.https://doi.org/10.1093/hmg/ddaa047
-
Deciphering OPA1 mutations pathogenicity by combined analysis of human, mouse and yeast cell modelsBiochimica et Biophysica Acta. Molecular Basis of Disease 1864:3496–3514.https://doi.org/10.1016/j.bbadis.2018.08.004
-
Processing of Mgm1 by the rhomboid-type protease Pcp1 is required for maintenance of mitochondrial morphology and of mitochondrial DNAThe Journal of Biological Chemistry 278:27781–27788.https://doi.org/10.1074/jbc.M211311200
-
Autophagy controls the pathogenicity of OPA1 mutations in dominant optic atrophyJournal of Cellular and Molecular Medicine 21:2284–2297.https://doi.org/10.1111/jcmm.13149
-
Basal mitophagy is widespread in Drosophila but minimally affected by loss of Pink1 or parkinThe Journal of Cell Biology 217:1613–1622.https://doi.org/10.1083/jcb.201801044
-
Dominant optic atrophy: Culprit mitochondria in the optic nerveProgress in Retinal and Eye Research 83:100935.https://doi.org/10.1016/j.preteyeres.2020.100935
-
Mitochondrial dynamics in mammalian health and diseasePhysiological Reviews 89:799–845.https://doi.org/10.1152/physrev.00030.2008
-
Chchd2 regulates mitochondrial morphology by modulating the levels of Opa1Cell Death and Differentiation 27:2014–2029.https://doi.org/10.1038/s41418-019-0482-7
-
OPA1 processing in cell death and disease - the long and short of itJournal of Cell Science 129:2297–2306.https://doi.org/10.1242/jcs.159186
-
An antibody toolbox to track complex I assembly defines AIF’s mitochondrial functionThe Journal of Cell Biology 219:e202001071.https://doi.org/10.1083/jcb.202001071
-
Mitochondrial dynamics and disease, OPA1Biochimica et Biophysica Acta 1763:500–509.https://doi.org/10.1016/j.bbamcr.2006.04.003
-
Polymorphisms in OPA1 are associated with normal tension glaucomaMolecular Vision 9:460–464.
-
EMBOSS: The european molecular biology open software suiteTrends in Genetics 16:276–277.https://doi.org/10.1016/s0168-9525(00)02024-2
-
A quantitative model of sporadic axonal degeneration in the Drosophila visual systemThe Journal of Neuroscience 42:4937–4952.https://doi.org/10.1523/JNEUROSCI.2115-21.2022
-
The BCL-2-like protein CED-9 of C. elegans promotes FZO-1/Mfn1,2- and EAT-3/Opa1-dependent mitochondrial fusionThe Journal of Cell Biology 186:525–540.https://doi.org/10.1083/jcb.200905070
-
Phospholipid association is essential for dynamin-related protein Mgm1 to function in mitochondrial membrane fusionThe Journal of Biological Chemistry 284:28682–28686.https://doi.org/10.1074/jbc.M109.044933
-
Early-onset severe neuromuscular phenotype associated with compound heterozygosity for OPA1 mutationsMolecular Genetics and Metabolism 103:383–387.https://doi.org/10.1016/j.ymgme.2011.04.018
-
Fiji: an open-source platform for biological-image analysisNature Methods 9:676–682.https://doi.org/10.1038/nmeth.2019
-
A novel mutation in the OPA1 gene in A Japanese patient with optic atrophyAmerican Journal of Ophthalmology 135:256–257.https://doi.org/10.1016/S0002-9394(02)01929-3
-
Analyzing synaptic modulation of Drosophila melanogaster photoreceptors after exposure to prolonged lightJournal of Visualized Experiments 1:55176.https://doi.org/10.3791/55176
-
Spectrum, frequency and penetrance of OPA1 mutations in dominant optic atrophyHuman Molecular Genetics 10:1369–1378.https://doi.org/10.1093/hmg/10.13.1369
-
Mitochondrial dysfunction induces dendritic loss via eIF2α phosphorylationThe Journal of Cell Biology 216:815–834.https://doi.org/10.1083/jcb.201604065
-
Axon degeneration: molecular mechanisms of a self-destruction pathwayThe Journal of Cell Biology 196:7–18.https://doi.org/10.1083/jcb.201108111
-
Rhomboid-7 and HtrA2/Omi act in a common pathway with the Parkinson’s disease factors Pink1 and ParkinDisease Models & Mechanisms 1:168–174.https://doi.org/10.1242/dmm.000109
-
Heterozygous loss-of-function DHX9 variants are associated with neurodevelopmental disorders: Human genetic and experimental evidencesEuropean Journal of Medical Genetics 66:104804.https://doi.org/10.1016/j.ejmg.2023.104804
-
OPA1-Exon4b Binds to mtDNA D-Loop for transcriptional and metabolic modulation, independent of mitochondrial fusionFrontiers in Cell and Developmental Biology 8:180.https://doi.org/10.3389/fcell.2020.00180
-
OPA1 increases the risk of normal but not high tension glaucomaJournal of Medical Genetics 47:120–125.https://doi.org/10.1136/jmg.2009.067512
-
A multiple sclerosis-like disorder in patients with OPA1 mutationsAnnals of Clinical and Translational Neurology 3:723–729.https://doi.org/10.1002/acn3.323
-
Sustained intracellular calcium rise mediates neuronal mitophagy in models of autosomal dominant optic atrophyCell Death and Differentiation 29:167–177.https://doi.org/10.1038/s41418-021-00847-3
Article and author information
Author details
Funding
Japan Society for the Promotion of Science (18K14835)
- Yohei Nitta
Japan Society for the Promotion of Science (18J00367)
- Yohei Nitta
Japan Society for the Promotion of Science (21K15619)
- Yohei Nitta
Japan Society for the Promotion of Science (21K06184)
- Satoko Hakeda-Suzuki
Japan Society for the Promotion of Science (16H06457)
- Takashi Suzuki
Japan Society for the Promotion of Science (21H02483)
- Takashi Suzuki
Japan Society for the Promotion of Science (21H05682)
- Takashi Suzuki
Japan Society for the Promotion of Science (17H04983)
- Atsushi Sugie
Japan Society for the Promotion of Science (19K22592)
- Atsushi Sugie
Japan Society for the Promotion of Science (21H02837)
- Atsushi Sugie
Japan Society for the Promotion of Science (24K02349)
- Atsushi Sugie
Japan Society for the Promotion of Science (24K22104)
- Atsushi Sugie
Japan Agency for Medical Research and Development (JP22ek019484s)
- Atsushi Sugie
Japan Agency for Medical Research and Development (JP24ek0109760s4001)
- Atsushi Sugie
NIG-JOINT
- Emiko Suzuki
Takeda Science Foundation (Visionary Research Grant)
- Takashi Suzuki
Takeda Science Foundation (Life Science Research Grant)
- Atsushi Sugie
Takeda Science Foundation (Bioscience Research Grant)
- Atsushi Sugie
GSK Japan Research Grant 2022 (AS2021A000166849)
- Atsushi Sugie
The funders had no role in study design, data collection and interpretation, or the decision to submit the work for publication.
Acknowledgements
We would like to thank Dr. Uemura for providing us with the transgenic fly strain and Dr. Owusu-Ansah for gifting us the antibody. The Ministry of Education, Culture, Sports, Science, and Technology of Japan (#18K14835, #18J00367, and #21K15619 to YN, #21K06184 to SHS, #16H06457, #21H02483, and 21H05682 to TS, #17H04983, #19K22592, and #21H02837 to AS), the Japan Agency for Medical Research and Development (AMED) (#JP22ek019484s) to AS, NIG-JOINT to ES, Takeda Science Foundation Takeda Visionary Research Grant to TS, Takeda Science Foundation Life Science Research Grant to AS, and GSK Japan Research Grant 2022 (AS2021A000166849) to AS.
Version history
- Preprint posted:
- Sent for peer review:
- Reviewed Preprint version 1:
- Reviewed Preprint version 2:
- Version of Record published:
Cite all versions
You can cite all versions using the DOI https://doi.org/10.7554/eLife.87880. This DOI represents all versions, and will always resolve to the latest one.
Copyright
© 2023, Nitta, Osaka et al.
This article is distributed under the terms of the Creative Commons Attribution License, which permits unrestricted use and redistribution provided that the original author and source are credited.
Metrics
-
- 1,153
- views
-
- 102
- downloads
-
- 0
- citations
Views, downloads and citations are aggregated across all versions of this paper published by eLife.
Download links
Downloads (link to download the article as PDF)
Open citations (links to open the citations from this article in various online reference manager services)
Cite this article (links to download the citations from this article in formats compatible with various reference manager tools)
Further reading
-
- Immunology and Inflammation
- Medicine
Preeclampsia (PE), a major cause of maternal and perinatal mortality with highly heterogeneous causes and symptoms, is usually complicated by gestational diabetes mellitus (GDM). However, a comprehensive understanding of the immune microenvironment in the placenta of PE and the differences between PE and GDM is still lacking. In this study, cytometry by time of flight indicated that the frequencies of memory-like Th17 cells (CD45RA−CCR7+IL-17A+CD4+), memory-like CD8+ T cells (CD38+CXCR3−CCR7+Helios−CD127−CD8+) and pro-inflam Macs (CD206−CD163−CD38midCD107alowCD86midHLA-DRmidCD14+) were increased, while the frequencies of anti-inflam Macs (CD206+CD163−CD86midCD33+HLA-DR+CD14+) and granulocyte myeloid-derived suppressor cells (gMDSCs, CD11b+CD15hiHLA-DRlow) were decreased in the placenta of PE compared with that of normal pregnancy (NP), but not in that of GDM or GDM&PE. The pro-inflam Macs were positively correlated with memory-like Th17 cells and memory-like CD8+ T cells but negatively correlated with gMDSCs. Single-cell RNA sequencing revealed that transferring the F4/80+CD206− pro-inflam Macs with a Folr2+Ccl7+Ccl8+C1qa+C1qb+C1qc+ phenotype from the uterus of PE mice to normal pregnant mice induced the production of memory-like IL-17a+Rora+Il1r1+TNF+Cxcr6+S100a4+CD44+ Th17 cells via IGF1–IGF1R, which contributed to the development and recurrence of PE. Pro-inflam Macs also induced the production of memory-like CD8+ T cells but inhibited the production of Ly6g+S100a8+S100a9+Retnlg+Wfdc21+ gMDSCs at the maternal–fetal interface, leading to PE-like symptoms in mice. In conclusion, this study revealed the PE-specific immune cell network, which was regulated by pro-inflam Macs, providing new ideas about the pathogenesis of PE.
-
- Medicine
Background: Several fields have described low reproducibility of scientific research and poor accessibility in research reporting practices. Although previous reports have investigated accessible reporting practices that lead to reproducible research in other fields, to date, no study has explored the extent of accessible and reproducible research practices in cardiovascular science literature.
Methods: To study accessibility and reproducibility in cardiovascular research reporting, we screened 639 randomly selected articles published in 2019 in three top cardiovascular science publications: Circulation, the European Heart Journal, and the Journal of the American College of Cardiology (JACC). Of those 639 articles, 393 were empirical research articles. We screened each paper for accessible and reproducible research practices using a set of accessibility criteria including protocol, materials, data, and analysis script availability, as well as accessibility of the publication itself. We also quantified the consistency of open research practices within and across cardiovascular study types and journal formats.
Results: We identified that fewer than 2% of cardiovascular research publications provide sufficient resources (materials, methods, data, and analysis scripts) to fully reproduce their studies. Of the 639 articles screened, 393 were empirical research studies for which reproducibility could be assessed using our protocol, as opposed to commentaries or reviews. After calculating an accessibility score as a measure of the extent to which an article makes its resources available, we also showed that the level of accessibility varies across study types with a score of 0.08 for Case Studies or Case Series and 0.39 for Clinical Trials (p = 5.500E-5) and across journals (0.19 through 0.34, p = 1.230E-2). We further showed that there are significant differences in which study types share which resources.
Conclusion: Although the degree to which reproducible reporting practices are present in publications varies significantly across journals and study types, current cardiovascular science reports frequently do not provide sufficient materials, protocols, data, or analysis information to reproduce a study. In the future, having higher standards of accessibility mandated by either journals or funding bodies will help increase the reproducibility of cardiovascular research.
Funding: Authors Gabriel Heckerman, Arely Campos-Melendez, and Chisomaga Ekwueme were supported by an NIH R25 grant from the National Heart, Lung and Blood Institute (R25HL147666). Eileen Tzng was supported by an AHA Institutional Training Award fellowship (18UFEL33960207).