Repurposing the mammalian RNA-binding protein Musashi-1 as an allosteric translation repressor in bacteria
Figures
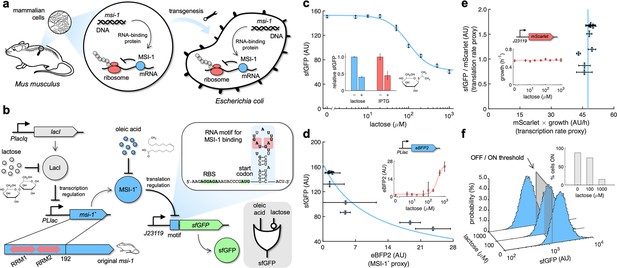
Musashi-1 can downregulate translation in bacteria.
(a) Overview of the biotechnological development. In mammals, MSI-1 binds to the 3’ untranslated region (UTR) of its target mRNA to repress translation. Here, the M. musculus gene coding for MSI-1 was moved to E. coli (transgenesis) to implement a synthetic regulation system at the level of translation. (b) Schematic of the synthetic gene circuit engineered in E. coli. A truncated version of MSI-1 (termed MSI-1*) was expressed from the PLlac promoter to be induced with lactose (or isopropyl β-D-1-thiogalactopyranoside [IPTG]) in a genetic background overexpressing LacI. sfGFP was used as a reporter expressed from a constitutive promoter (J23119) and under the control of a suitable RNA motif recognized by MSI-1* in the N-terminal coding region of the transcript (viz., located after the start codon). The activity of MSI-1* could in turn be allosterically inhibited by oleic acid. In electronic terms, this circuit implements an IMPLY logic gate. The inset shows the predicted secondary structure of the N-terminal coding region of the reporter mRNA. Within the motif (blue shaded), the consensus recognition sequences (RUnAGU) are bolded and the minimal cores (UAG) are marked in red. System implemented with pRM1+ and pREP6. (c) Dose–response curve of the system using lactose as inducer (up to 1 mM). MSI-1* downregulated sfGFP expression by 2.5-fold. The inset shows the dynamic range of the response using lactose or IPTG (1 mM), showing a statistically significant regulation in both cases (Welch’s t-test, two-tailed p<0.05). (d) Transfer function of the system (between sfGFP and MSI-1*). The inset shows the dose–response curve of eBFP2 expressed from the PLlac promoter (proxy of MSI-1* expression) with lactose. (e) Scatter plot of the dynamic response of the system in the Crick space (translation rate vs. transcription rate). The dose–response curve of mScarlet expressed from the J23119 promoter with lactose was used to perform the decomposition (vertical line fitted to 48 AU/h). The inset shows the growth rate of the cells for each induction condition (horizontal line fitted to 0.55 h–1). In all cases, points correspond to experimental data, while solid lines come from adjusted mathematical models. Error bars correspond to standard deviations (n = 3). (f) Probability-based histograms of sfGFP expression from single-cell data for different lactose concentrations, showing a statistically significant regulation (one-way ANOVA, p<10–4). The inset shows the percentage of cells in the ON state (sfGFP expressed), according to a specified threshold, for each lactose concentration. AU, arbitrary units.
-
Figure 1—source data 1
Bulk fluorescence data of sfGFP, eBFP2, and mScarlet with lactose and single-cell data of sfGFP.
- https://cdn.elifesciences.org/articles/91777/elife-91777-fig1-data1-v1.xlsx
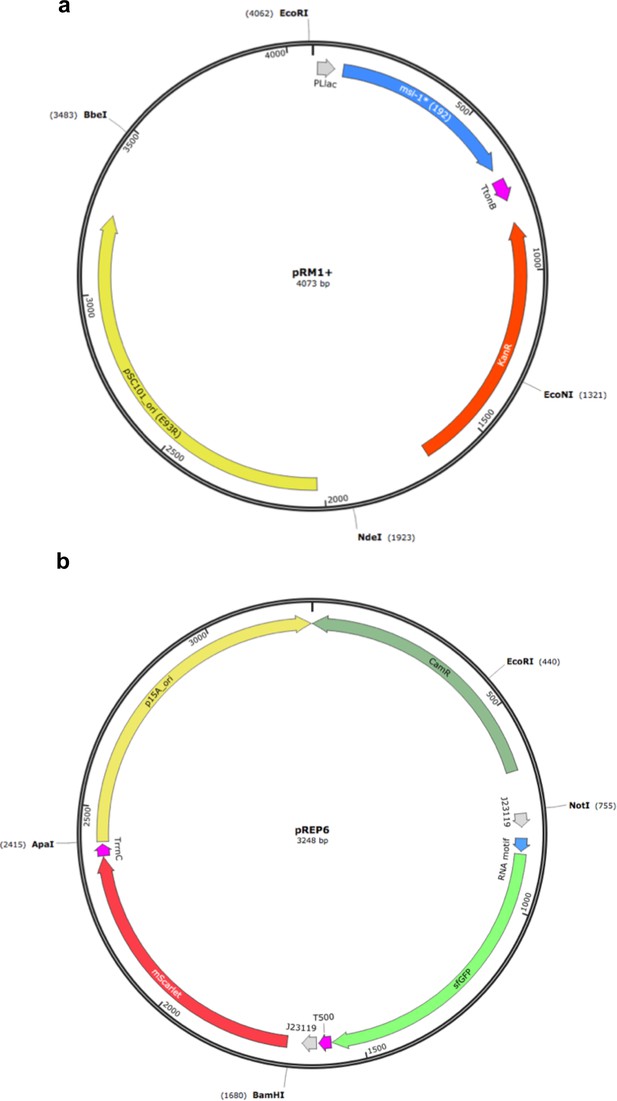
Maps of the plasmids used to implement the synthetic gene circuit in which MSI-1* represses the translation of sfGFP.
(a) Map of pRM1+ to express the MSI-1* protein from a PLlac promoter, induced with lactose or IPTG. (b) Map of pREP6 to express the reporter sfGFP protein from a constitutive promoter (J23119), harboring a suitable RNA motif in the leader region for translation regulation.

RT-qPCR results for the mRNA level of sfGFP.
System implemented with pRM1+ and pREP6 or pREP7, induction with 1mM lactose, showing no significant change (Welch’s t-test, two-tailed p>0.05). Error bars correspond to standard deviations (n = 3). The oligonucleotide sequences used to amplify the housekeeping gene (in this case, b3500) were TGAGCATCTGGATTACAGCAAC (forward) and CGCGGTGAAAGAGGATTTATAC (reverse) and the probe was TAMRA-TCCGCCGATTGGTACTGTTGGTTT-Q, where the quencher Q was Iowa Black FQ. The oligonucleotide sequences used to amplify sfGFP were GTTCAGTGCTTTGCTCGTTATC (forward) and GTACGTGCCGTCATCCTTAAA (reverse) and the probe was FAM-AGCATGACTTCTTCAAGTCCGCCA-Q.
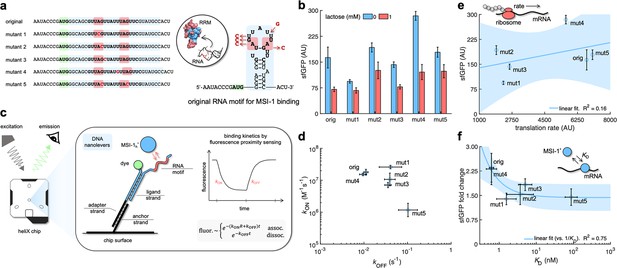
Mechanistic characterization of the Musashi-1–mRNA interaction.
(a) Sequences and predicted secondary structures of the different RNA motif variants for MSI-1 binding analyzed in this work. Point-mutations indicated in red. Three-dimensional representations of the RRM1 and RNA motif are also shown. Within the RRM1, the region that recognizes the RNA is shown in blue. (b) Dynamic range of the response of the different genetic systems using lactose (1 mM), showing a statistically significant regulation in all cases (Welch’s t-test, two-tailed p<0.05; although some mutants present a small fold change). (c) Schematic of the heliX biosensor platform. A double-strand DNA nanolever was immobilized on a gold electrode of the chip. The nanolever carried a fluorophore in one end and the RNA motif for MSI-1 binding in the other. Binding between MSI-1h* (injected analyte) and RNA led to a fluorescence change, whose monitoring in real time served to extract the kinetic constants that characterize the interaction. (d) Scatter plot of the experimentally-determined kinetic constants of association and dissociation between the protein and the RNA for all systems (original and five mutants). Means and deviations calculated in log scale (geometric). (e) Correlation between the maximal sfGFP expression level (in the absence of lactose) and the translation rate predicted with RBS calculator. Linear regression performed. (f) Correlation between the fold change in sfGFP expression and the dissociation constant (KD). Deviations calculated by propagation. Linear regression performed (vs. 1/KD). Blue shaded areas indicate 95% confidence intervals. In all cases, error bars correspond to standard deviations (n = 3). AU, arbitrary units.
-
Figure 2—source data 1
Bulk fluorescence data of sfGFP and binding kinetics measurements.
- https://cdn.elifesciences.org/articles/91777/elife-91777-fig2-data1-v1.xlsx
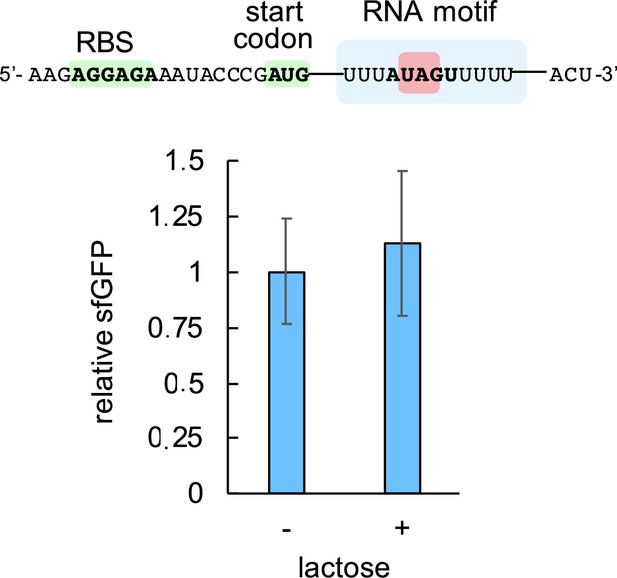
Characterization of the system response with lactose using pREP4 as a reporter plasmid.
Induction with 1 mM lactose, showing no significant regulation (Welch’s t-test, two-tailed p>0.05). Error bars correspond to standard deviations (n = 3). On the top, sequence of the leader RNA of sfGFP in this case. While MSI-1* can bind to this sequence in vitro, these data show that an efficient interaction in vivo requires two copies of the consensus recognition sequence for the two RRMs.

Musashi protein purification.
(a) Schematics of the experimental procedure for MSI-1h* purification. E. coli cells expressing MSI-1h* were lysed, nucleic acids were removed, and the protein was purified by ion exchange chromatography. (b) Gel electrophoretic assay to confirm the presence of MSI-1h*. In particular, this was a sodium dodecyl sulfate polyacrylamide gel electrophoresis (SDS-PAGE). M, molecular marker (Pierce unstained protein MW marker, 14.4–116 kDa, Thermo). (c) Sequence comparison between the human and the mouse MSI-1 proteins. The only different residue is located in the first helix of the RRM2. It is residue 127, a histidine in mice and glutamine in humans. This is not expected to make a significant difference in structure or function. The protein that was purified is the human version (denoted by MSI-1h*), while the protein that implements the regulatory circuit is the mouse version (denoted by MSI-1*). (d) Three-dimensional structural schematic of the RRM2 of mouse MSI-1.

Characterization of different mutant RNA motifs in terms of binding kinetics against the MSI-1h* protein.
(a) Predicted secondary structures of original and mutant RNA motifs; mutations are marked in red. (b) Binding and unbinding kinetic curves for the different RNA sequences (representative samples). The kinetic constants were extracted from mono-exponential model fits. For the original, mutant 1, mutant 2, and mutant 4 RNA motifs, a bi-exponential model was also explored to describe the binding kinetics, reflecting two types of interactions, although with little improvement. (c) Inferred kinetic constants (kON, kOFF) and the resulting dissociation constant (KD) for each sequence and replicate.
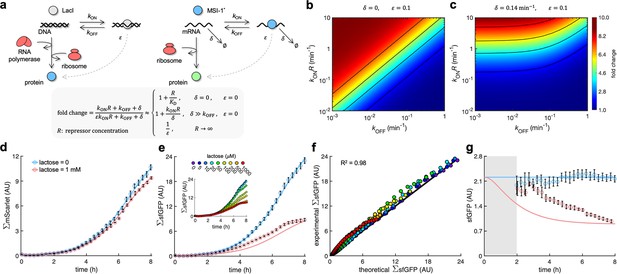
A mathematical model captures the dynamic response of the system.
(a) Schematics of gene regulation at different levels with proteins that bind to nucleic acids (DNA or RNA). On the left, schematic of transcription regulation (e.g., LacI regulating MSI-1* expression). On the right, schematic of translation regulation (e.g., MSI-1* regulating sfGFP expression). A general mathematical expression (gray shaded) was derived to calculate the fold change in protein expression as a function of the regulator concentration (R), the association and dissociation rates (kON and kOFF), the elongation leakage fraction (), and the nucleic acid degradation rate (). (b) Heatmap of the fold change as a function of kONR and kOFF (i.e., the first-order kinetic rates that characterize the protein–DNA/RNA interaction) when and . This would correspond to transcription regulation. (c) Heatmap of the fold change when min–1 and . This would correspond to translation regulation. (d) Total red fluorescence of the cell population (ΣmScarlet) over time without and with 1 mM lactose. In this case, the cell growth rate was fitted to 0.80 h–1. (e) Total green fluorescence of the cell population (ΣsfGFP) over time without and with 1 mM lactose. The inset shows the dynamic response for different lactose concentrations. (f) Correlation between the experimental values of ΣsfGFP at different times and for different lactose concentrations and the predicted values from a mathematical model that accounts for population growth and gene regulation. Data for t > 2 h. Linear regression performed. (g) Ratio of total green and red fluorescence as a proxy of cellular sfGFP expression over time. Ratio not represented at early times due to the high error obtained given the low number of cells present in the culture (gray shaded area). Deviations calculated by propagation. In all cases, points correspond to experimental data, while solid lines come from an adjusted mathematical model. Error bars correspond to standard deviations (n = 3). AU, arbitrary units.
-
Figure 3—source data 1
Bulk fluorescence data of sfGFP and mScarlet with time.
- https://cdn.elifesciences.org/articles/91777/elife-91777-fig3-data1-v1.xlsx
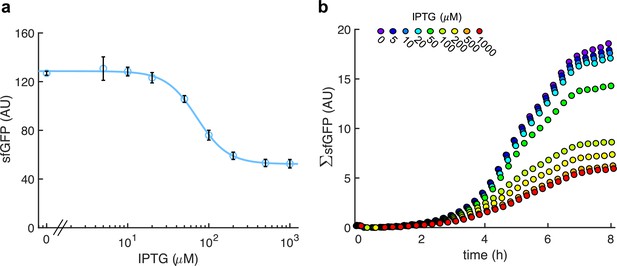
Characterization of the system response with IPTG (implemented with pRM1+ and pREP6).
(a) Dose–response curve. Error bars correspond to standard deviations (n = 3). (b) Time-course response for different inducer concentrations (average of four clones). The fluorescence of the whole population is represented (ΣsfGFP).
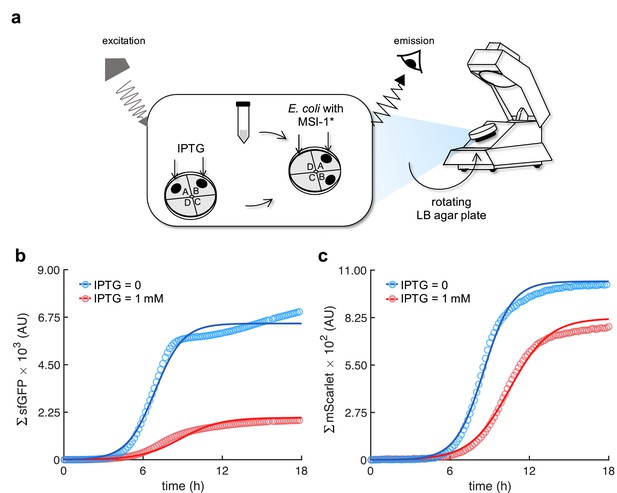
Dynamic response of the system in solid medium.
(a) Schematics of the LigandTracer technology repurposed for characterizing bacterial cells expressing fluorescent proteins (implemented with pRM1+ and pREP6). (b) Real-time green fluorescence of the whole population (ΣsfGFP) upon induction with IPTG. (c) Real-time red fluorescence (ΣmScarlet). Points correspond to the experimental data, while solid lines come from an adjusted mathematical model.
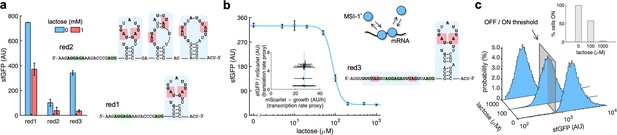
mRNA redesign to enhance the downregulation by Musashi-1.
(a) Dynamic range of the response of three redesigned genetic systems using lactose (1 mM), showing a statistically significant regulation in the first and third cases (Welch’s t-test, two-tailed p<0.05). The predicted secondary structures of the N-terminal coding regions of the reporter mRNAs are shown on the right. Redesign 1 (red1) was implemented with pREP4b and redesign 2 (red2) with pREP4b3x, which contains three MSI-1 binding sites. These stem-loop structures are less stable than the original one. Redesign 3 (red3) was implemented with pREP7. (b) Dose–response curve of the redesign-3 system using lactose as inducer (up to 1 mM). MSI-1* downregulated sfGFP expression by 8.6-fold (Welch’s t-test, two-tailed p<0.05). The inset shows the scatter plot of the dynamic response in the Crick space (translation rate vs. transcription rate; vertical line fitted to 27 AU/h). The predicted secondary structure of the N-terminal coding region of the reporter mRNA is shown on the right; the mRNA contains two MSI-1 binding sites (blue shaded). In the 5’ UTR, the binding site is formed by two RUnAGU repeats that flank the RBS without forming secondary structure. In the N-terminal coding region, the binding site is the original one. The minimal cores (UAG) are marked in red. Points correspond to experimental data, while the solid line comes from an adjusted mathematical model. In all cases, error bars correspond to standard deviations (n = 3). (c) Probability-based histograms of sfGFP expression from single-cell data for different lactose concentrations (redesign 3), showing a statistically significant regulation (one-way ANOVA, p<10–4). The inset shows the percentage of cells in the ON state (sfGFP expressed), according to a specified threshold, for each lactose concentration. AU, arbitrary units.
-
Figure 4—source data 1
Bulk fluorescence data of sfGFP with lactose.
- https://cdn.elifesciences.org/articles/91777/elife-91777-fig4-data1-v1.xlsx
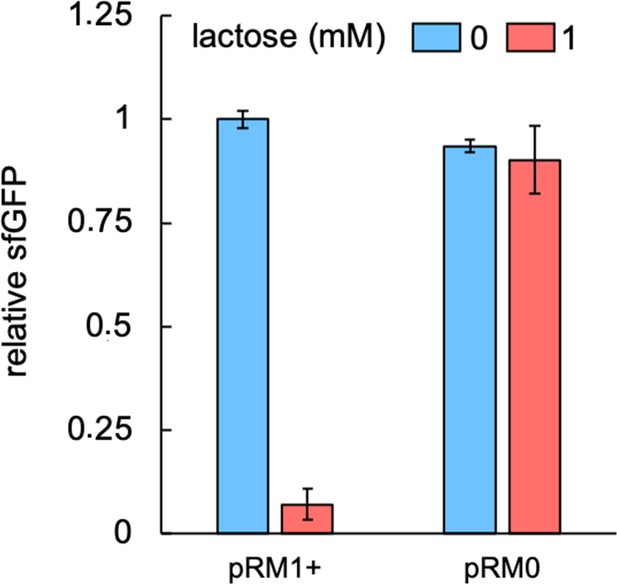
PLlac promoter tightly controls MSI-1* expression.
Characterization of the response with lactose using pREP7 as a reporter plasmid (induction with 1 mM lactose), showing similar fluorescence values in the absence of lactose with and without msi-1*, as well as no significant fluorescence change with lactose in the absence of msi-1*. Error bars correspond to standard deviations (n = 3). pRM0 is a void plasmid that does not harbor the msi-1* genetic cassette.
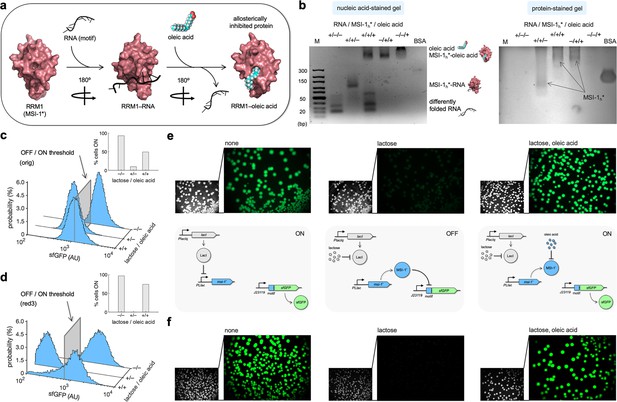
Oleic acid inhibits the regulatory activity of Musashi-1 in bacteria.
(a) Three-dimensional structural schematic of the allosteric regulation. RRM1 of MSI-1 is shown alone, in complex with the RNA motif, and in complex with oleic acid. (b) Gel electrophoresis mobility shift assay to test the allosteric inhibition of MSI-1* with oleic acid. A purified MSI-1* protein (45 μM), the RNA motif as a label-free sRNA molecule (11 μM), and oleic acid (1 mM) were mixed in a combinatorial way in vitro. On the left, nucleic acid-stained gel. On the right, protein-stained gel (Coomassie). The different formed species are indicated. M denotes molecular marker (GeneRuler ultra-low range DNA ladder, 10–300 bp, Thermo). BSA was used as a control. (c, d) Probability-based histograms of sfGFP expression from single-cell data for different induction conditions (1 mM lactose or 1 mM lactose + 20 mM oleic acid) for the original system (c) and the redesign-3 system (d), showing statistically significant regulation in both cases (one-way ANOVA, p<10–4). The insets show the percentages of cells in the ON state (sfGFP expressed), according to a specified threshold, for each condition. (e) On the top, images of E. coli colonies harboring pRM1+ and pREP6. Bacteria were seeded in LB-agar plates with suitable inducers (1 mM lactose or 1 mM lactose + 20 mM oleic acid). Fluorescence and bright-field images are shown. On the bottom, schematics of the working modes of the synthetic gene circuit according to the different induction conditions. (f) Images of E. coli colonies harboring pRM1+ and pREP7. AU, arbitrary units.
-
Figure 5—source data 1
Single-cell data of sfGFP.
- https://cdn.elifesciences.org/articles/91777/elife-91777-fig5-data1-v1.xlsx
-
Figure 5—source data 2
Full gel images.
- https://cdn.elifesciences.org/articles/91777/elife-91777-fig5-data2-v1.zip

Gel electrophoresis mobility shift assays to test the MSI-1h*-RNA and the MSI-1h*-oleic acid interactions (nucleic acid-stained gels).
(a) Interaction of MSI-1h* with the RNA motif. RNA added at 11 μM. (b) Interaction of MSI-1h* with oleic acid. RNA added at 11 μM and MSI-1h* at 45 μM. M, molecular marker (GeneRuler ultra-low range DNA ladder, 10–300 bp, Thermo).
-
Figure 5—figure supplement 1—source data 1
Full gel images.
- https://cdn.elifesciences.org/articles/91777/elife-91777-fig5-figsupp1-data1-v1.zip
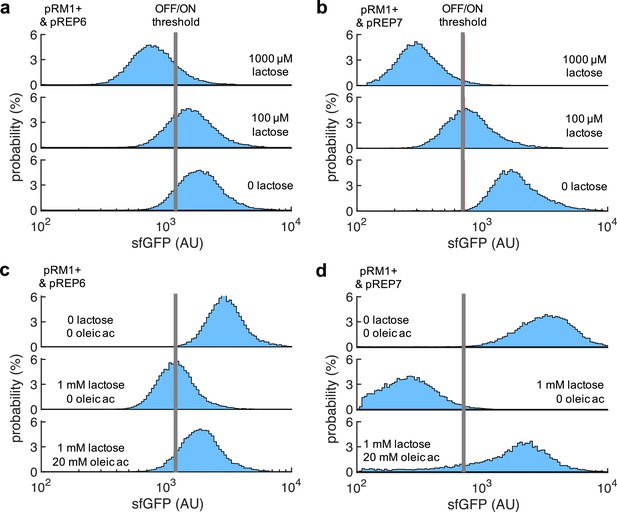
2D visualization of probability-based histograms of sfGFP expression from single-cell data.
(a) System implemented with pRM1+ and pREP6 in response to lactose. This corresponds to Figure 1f. (b) System implemented with pRM1+ and pREP7 in response to lactose. This corresponds to Figure 4c. (c) System implemented with pRM1+ and pREP6 in response to lactose and oleic acid. This corresponds to Figure 5c. (d) System implemented with pRM1+ and pREP7 in response to lactose and oleic acid. This corresponds to Figure 5d.
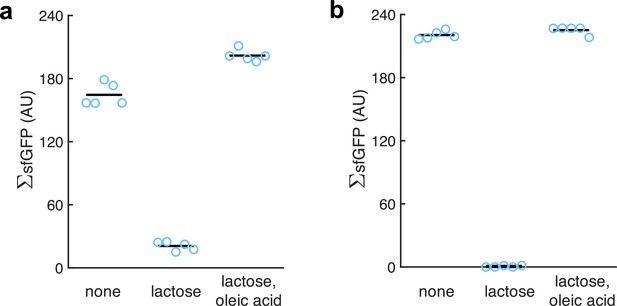
Quantification of the green fluorescence of the colonies (denoted by ΣsfGFP as it is from populations; n = 5).
(a) From images of E. coli colonies harboring pRM1+ and pREP6. (b) From images of E. coli colonies harboring pRM1+ and pREP7. Oleic acid produced a statistically significant response in both cases (Welch’s t-test, two-tailed p<10-4).
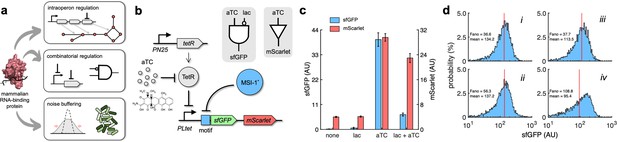
Applications of Musashi-1 for a fine expression control in bacteria.
(a) Overview of the regulatory utility of MSI-1*. It could (i) regulate the expression of a given enzyme belonging to a polycistronic operon for a metabolic pathway control, (ii) be exploited together with transcription factors to implement combinatorial regulations following the genetic information flow, envisioning biosensing applications, and (iii) regulate noise in protein expression with the aim of producing cell populations less disperse, especially for bacterial delivery applications in animals. (b) Schematic of a new synthetic gene circuit engineered in E. coli. MSI-1* was always expressed from the PLlac promoter to be induced with lactose in a genetic background overexpressing LacI. sfGFP and mScarlet were used as reporters, both expressed from the PLtet promoter to be induced with anhydrotetracycline (aTC) in a genetic background overexpressing TetR. In this bicistronic operon, only sfGFP was under the control of a suitable RNA motif recognized by MSI-1* in the leader region of the transcript (original motif or redesign-3). In electronic terms, this circuit implements a NIMPLY logic gate considering sfGFP as the output. System implemented with pRM1+ and pREP6α or pREP7α. (c) Dynamic range of the response using lactose (1 mM) and aTC (100 ng/mL) in a combinatorial way. aTC significantly activated the expression of the operon (Welch’s t-test, two-tailed p<0.05) and lactose, through the action of MSI-1*, significantly downregulated sfGFP expression in a specific way (data for pREP7α; Welch’s t-test, two-tailed p<0.05). Error bars correspond to standard deviations (n = 3). (d) Probability-based histograms of sfGFP expression from single-cell data for different inducer concentrations. On the left, pRM1+ and pREP6α with 100 ng/mL aTC and 1 mM lactose (i) or 15 ng/mL aTC (ii). On the right, pRM1+ and pREP7α with 100 ng/mL aTC and 1 mM lactose (iii) or 30 ng/mL aTC (iv). The mean expression and Fano factor are shown. AU, arbitrary units.
-
Figure 6—source data 1
Bulk fluorescence data of sfGFP and mScarlet and single-cell data of sfGFP.
- https://cdn.elifesciences.org/articles/91777/elife-91777-fig6-data1-v1.xlsx
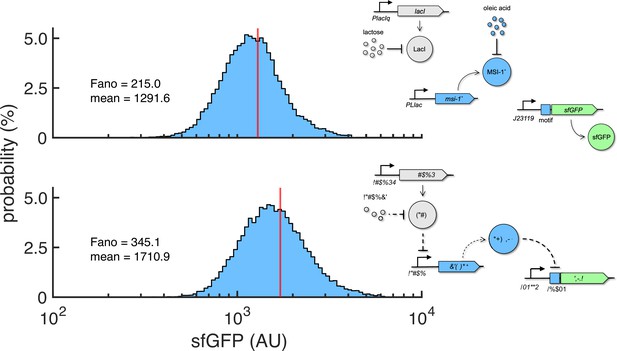
Probability-based histograms of sfGFP expression from single-cell data for different inducer concentrations (1 mM lactose + 20 mM oleic acid on the top, 0.1 mM lactose on the bottom).
The mean expression and Fano factor are shown. Implementation with pRM1+ and pREP6. Schematics of the working modes of the synthetic gene circuit are shown.