Circular RNA HMGCS1 sponges MIR4521 to aggravate type 2 diabetes-induced vascular endothelial dysfunction
eLife assessment
This study presents important findings linking circHMGCS1 and miR-4521 in diabetes-induced vascular endothelial dysfunction. Overall, the evidence supporting the claims of the authors is convincing. The work will be of interest to biomedical scientists working with cardiovascular and/or RNA biology, particularly those studying diabetes.
https://doi.org/10.7554/eLife.97267.3.sa0Important: Findings that have theoretical or practical implications beyond a single subfield
- Landmark
- Fundamental
- Important
- Valuable
- Useful
Convincing: Appropriate and validated methodology in line with current state-of-the-art
- Exceptional
- Compelling
- Convincing
- Solid
- Incomplete
- Inadequate
During the peer-review process the editor and reviewers write an eLife Assessment that summarises the significance of the findings reported in the article (on a scale ranging from landmark to useful) and the strength of the evidence (on a scale ranging from exceptional to inadequate). Learn more about eLife Assessments
Abstract
Noncoding RNA plays a pivotal role as novel regulators of endothelial cell function. Type 2 diabetes, acknowledged as a primary contributor to cardiovascular diseases, plays a vital role in vascular endothelial cell dysfunction due to induced abnormalities of glucolipid metabolism and oxidative stress. In this study, aberrant expression levels of circHMGCS1 and MIR4521 were observed in diabetes-induced human umbilical vein endothelial cell dysfunction. Persistent inhibition of MIR4521 accelerated development and exacerbated vascular endothelial dysfunction in diabetic mice. Mechanistically, circHMGCS1 upregulated arginase 1 by sponging MIR4521, leading to decrease in vascular nitric oxide secretion and inhibition of endothelial nitric oxide synthase activity, and an increase in the expression of adhesion molecules and generation of cellular reactive oxygen species, reduced vasodilation and accelerated the impairment of vascular endothelial function. Collectively, these findings illuminate the physiological role and interacting mechanisms of circHMGCS1 and MIR4521 in diabetes-induced cardiovascular diseases, suggesting that modulating the expression of circHMGCS1 and MIR4521 could serve as a potential strategy to prevent diabetes-associated cardiovascular diseases. Furthermore, our findings provide a novel technical avenue for unraveling ncRNAs regulatory roles of ncRNAs in diabetes and its associated complications.
Introduction
Cardiovascular disease (CVD) is the leading cause of morbidity and mortality in patients with type 2 diabetes mellitus (T2DM). The incidence of CVD in T2DM individuals was estimated to be at least two to four times higher than that in non-diabetic individuals (Gregg et al., 2016; Yun and Ko, 2021). T2DM gives rise to abnormal metabolic conditions, including chronic hyperglycemia, dyslipidemia, insulin resistance, inflammation, and oxidative stress (Roden and Shulman, 2019). These processes ultimately lead to vascular endothelial damage, which disrupts the maintenance of vascular homeostasis, thereby promoting the development of CVD (Lundberg and Weitzberg, 2022; Niemann et al., 2017). Vascular endothelial dysfunction (VED) is considered the underlying basis for vascular complications at all stages of T2DM (Eelen et al., 2015; Meigs et al., 2004). However, the mechanism by which the key molecules trigger endothelial dysfunction in diabetes-induced vascular impairment remains unknown.
Endothelial cells form a continuous monolayer lining the arterial, venous, and lymphatic vessels, playing a crucial physiological role in maintaining vascular homeostasis (Bazzoni and Dejana, 2004). Moreover, endothelial cells actively regulate vascular tone, preserve endothelial integrity, and modulate platelet activity, contributing to hemostasis and endothelial repair (Li et al., 2019). The leading cause of T2DM is a prolonged high-sugar, high-fat diet (HFHG), commonly known as a Western diet, which inevitably leads to VED, characterized by reduced content of nitric oxide (NO), uncoupling of endothelial nitric oxide synthase (ENOS), increased formation of reactive oxygen species (ROS) and upregulation of endothelial-related adhesion molecules such as intercellular adhesion molecule 1 (ICAM1), vascular cell adhesion molecule 1 (VCAM1), and endothelin 1 (ET-1). These alterations ultimately lead to elevated blood pressure and impaired vascular arteriolar relaxation, contributing to CVD development (Xu et al., 2021; Yeh et al., 2022; Zheng et al., 2018). Despite this understanding, the identification of pivotal regulatory factors governing gene expression in diabetes-induced VED remains a formidable challenge.
circRNAs are generated through back-splicing of precursor messenger RNAs (pre-mRNAs), exhibiting features such as a covalently closed loop structure, high stability, conservation, and tissue-specific expression. These molecules primarily function as miRNA sponges, regulators of transcription, and interactors with proteins (Kristensen et al., 2019; Liu and Chen, 2022). Conversely, miRNAs are single-stranded small RNA molecules of 21–23 nucleotides, characterized by high conservation, temporal and tissue-specific expression, and relatively poor stability. miRNAs—critical regulators of gene expression—control a wide array of cellular processes (Gebert and MacRae, 2019). The interplay between miRNAs and circRNAs forms a complex regulatory network, profoundly influencing diverse biological pathways and disease states. miRNAs serve as guides, targeting specific mRNAs to induce their degradation or translational repression, thereby suppressing gene expression post-transcriptionally (Treiber et al., 2019). By contrast, circRNAs contain miRNA binding sites, competitively inhibiting miRNA activity and thereby reducing miRNA binding to other mRNAs. This competitive adsorption effect modulates intracellular miRNA levels, affecting miRNA-mediated regulation of other target genes (Cheng et al., 2019b; Huang et al., 2019; Shen et al., 2019; Zeng et al., 2021).
The expression levels of circRNA in diabetic patients exhibit abnormalities, and its potential as a biomarker for early diagnosis or therapeutic target has been gradually being substantiated (Jiang et al., 2022; Stoll et al., 2020; Tian et al., 2018). However, research on circRNA in the context of diabetes-induced VED is scarce. Current research has predominantly focused on the regulatory roles of circRNA in diabetic cardiomyopathy (Yuan et al., 2023), diabetic nephropathy (Yang and Liu, 2022), gestational diabetes (Du et al., 2022), and diabetic retinopathy (Zhu et al., 2019). Investigations into circRNA regulation of VED have only described the phenomenon and analyzed the correlation of the results, lacking the precision to infer causation accurately (Jiang et al., 2020; Liu et al., 2017; Ma et al., 2023). Moreover, comprehension of VED induced by the complex multifactorial processes of diabetes pathogenesis remains relatively inadequate. Additionally, the interaction between circRNA and miRNA in diabetes-induced VED remains a subject of research controversy (Cheng et al., 2019a; Liu et al., 2019; Pan et al., 2018). Therefore, a more comprehensive research approach is required to elucidate the specific mechanisms by which circRNA operates in diabetes-related CVDs.
The present study elucidated the mechanisms underlying the interaction between circRNA and miRNA in regulating diabetes-associated VED. Through the screening strategy, we successfully identified circHMGCS1—a circRNA originating from the pre-mRNA of HMGCS1. We demonstrated that the upregulation of circHMGCS1 and downregulation of MIR4521 significantly promoted diabetes-induced VED. Moreover, in-depth mechanistic investigations revealed that circHMGCS1 functioned as a MIR4521 sponge, increasing arginase 1 (ARG1) expression in vascular endothelial cells and accelerating VED progression. Furthermore, the expression patterns and interaction mechanisms between circHMGCS1 and MIR4521 in endothelial cells were identified for the first time in these findings, which also highlight the circHMGCS1/MIR4521/ARG1 axis as a novel therapeutic target for interventions designed to protect patients from diabetes-induced VED.
Results
Global expression analysis of endotheliocyte circRNAs
To explore the potential role of circRNAs in regulating endotheliocyte function, we characterized the transcriptome of circRNA derived from total RNA in human umbilical vein endothelial cells (HUVECs) stimulated by high palmitate and high glucose (PAHG), which allowed us to investigate the potential role of circRNAs in diabetes-induced VED (Figure 1—figure supplement 1A–C). A total of 17,179 known circRNAs were identified, with 66 of them exhibiting differential expression (GEO submission: GSE237597). Our main focus was on circRNAs that shared identical genomic and splicing lengths that exhibited fold changes greater than 2 or less than –2 (p<0.01) in PAHG-treated HUVECs (Figure 1A). Specifically, 48 circRNAs were upregulated, whereas 18 were downregulated (Figure 1—figure supplement 1D). Furthermore, approximately 66.65% of circRNAs were found to be produced through reverse splicing of exons (Figure 1—figure supplement 1E).
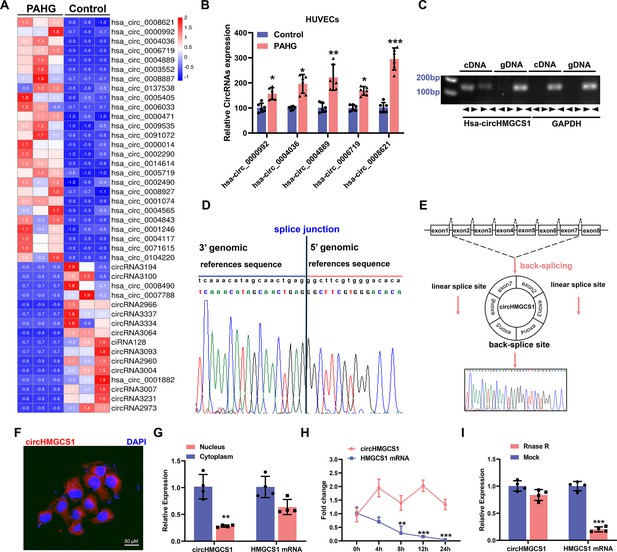
circHMGCS1 upregulation and its association with PAHG-induced endothelial dysfunction.
(A) Heat map illustrating the differential expression of circRNAs in HUVECs treated with PAHG for 24 hours (n=3). (B) qRT-PCR validation of five circRNAs in HUVECs treated with PAHG compared to normal cells, normalized to GAPDH (n=6). (C) PCR validation of circHMGCS1 presence in HUVECs, GAPDH was utilized as a negative control (n=6). (D) Alignment of circHMGCS1 sequence in CircBase (circular RNA database, upper) in agreement with Sanger sequencing results (lower). (E) Schematic representation of circularization of exons 2–7 of HMGCS1 (red arrow). (F) RNA-FISH analysis detecting circHMGCS1 expression in HUVECs using Cy3-labeled probes, Nuclei were counterstained with DAPI (red represents circHMGCS1, blue represents nucleus, scale bar=50 μm, n=4). (G) qRT-PCR quantification of circHMGCS1 and HMGCS1 mRNA levels in the cytoplasm or nucleus of HUVECs. circHMGCS1 and HMGCS1 mRNA levels were normalized to cytoplasmic values (n=4). (H) qRT-PCR measure circHMGCS1 and HMGCS1 mRNA levels after actinomycin D treatment (n=4 at different time points). (I) circHMGCS1 expression was detected in RNase R-treated total RNA by qRT-PCR (n=4). *p<0.05, **p<0.01, ***p<0.001, All significant difference was determined by unpaired two-tailed Student’s t-test or one-way ANOVA followed by Bonferroni multiple comparison post hoc test, error bar indicates SD.
-
Figure 1—source data 1
Uncropped and labeled gels for (Figure 1).
- https://cdn.elifesciences.org/articles/97267/elife-97267-fig1-data1-v1.zip
-
Figure 1—source data 2
Raw unedited gels for (Figure 1).
- https://cdn.elifesciences.org/articles/97267/elife-97267-fig1-data2-v1.zip
The observed changes in circRNA levels were confirmed through the real-time quantitative reverse transcription PCR (qRT-PCR) analysis of the five most upregulated circRNAs, suggesting that the results of the RNA-seq data are credible. Among them, circHMGCS1 (hsa_circ_0008621, 899) nt in length, identified as circHMGCS1 in subsequent studies because of its host gene being HMGCS1 (Figure 1—figure supplement 1F; Liang et al., 2021) exhibited significant upregulation compared with the non-PAHG-treated condition (Figure 1B). The circHMGCS1 sequence is situated on chromosome 5 of the human genome, specifically at 43292575–43297268 with no homology to mouse sequences. Subsequently, divergent primers were designed to amplify circHMGCS1, whereas convergent primers were designed to amplify the corresponding linear mRNA from cDNA and genomic DNA (gDNA) in HUEVCs. amplification of circHMGCS1 was observed in cDNA but not in gDNA (Figure 1C), which confirmed the circularity of circHMGCS1. Sanger sequencing of the amplified product of circHMGCS1 confirmed its sequence alignment with the annotated circHMGCS1 in circBase (Figure 1D). This observation confirms the origin of circHMGCS1 from exons 2–7 of the HMGCS1 gene (Figure 1E and Figure 1—figure supplement 1G, H).
RNA-Fluorescence in situ hybridization (RNA-FISH) confirmed the robust expression of cytoplasmic circHMGCS1 in HUVECs (Figure 1F). The qRT-PCR analysis of nuclear and cytoplasmic RNA revealed the predominant cytoplasmic expression of circHMGCS1 in HUVECs (Figure 1G). qRT-PCR also demonstrated that circHMGCS1 displayed a stable half-life exceeding 24 hr, whereas the linear transcript HMGCS1 mRNA had a half-life of less than 8 hr (Figure 1H). Furthermore, circHMGCS1 exhibited resistance to digestion by ribonuclease R (an exonuclease that selectively degrades linear RNA Xiao and Wilusz, 2019, also known as RNase R), whereas linear HMGCS1 mRNA was easily degraded upon RNase R treatment (Figure 1I). These findings indicate that the higher expression of circHMGCS1 in VED is more than just a byproduct of splicing and suggestive of functionality.
Upregulation of circHMGCS1 promotes diabetes-induced VED
To explore the potential role of circHMGCS1 in regulating endothelial cell function, we cloned exons 2–7 of HMGCS1 into lentiviral vectors for ectopic overexpression of circHMGCS1 (Figure 2—figure supplement 1). We found that circHMGCS1 was successfully overexpressed in HUVECs without significant changes in HMGCS1 mRNA expression, and the level of the circular transcripts increased nearly 60-fold than the level of the linear transcripts (Figure 2A). These results confirm that the circularization is efficient and that circHMGCS1 has no effect on HMGCS1 expression. Further experiments demonstrated that the overexpression of circHMGCS1 stimulated the expression of adhesion molecules (VCAM1, ICAM1, and ET-1; Figure 2B, C), suggesting that circHMGCS1 is involved in VED promotion. We then used PAHG to stimulate HUVEC-overexpressing circHMGCS1. NO levels were significantly decreased after PAHG stimulation for 24 hr, and circHMGCS1 overexpression further decreased NO levels (Figure 2D), indicating that increased circHMGCS1 expression inhibits endothelial diastolic function. ENOS activity was inhibited by PAHG, and upregulated circHMGCS1 expression further decreased ENOS activity (Figure 2E). Superoxide is one of the major ROS known to promote atherosclerosis (Griendling et al., 2016). Therefore, we used DHE to assess superoxide levels in endothelial cells. The overexpression of circHMGCS1 enhanced PAHG-induced ROS generation (Figure 2F), increasing oxidative stress in endothelial cells. The expression levels of adhesion molecules (VCAM1, ICAM1, and ET-1) were further elevated with circHMGCS1 overexpression (Figure 2G, H). These findings suggest that elevated expression of circHMGCS1 may contribute to the development of endothelial cell dysfunction.
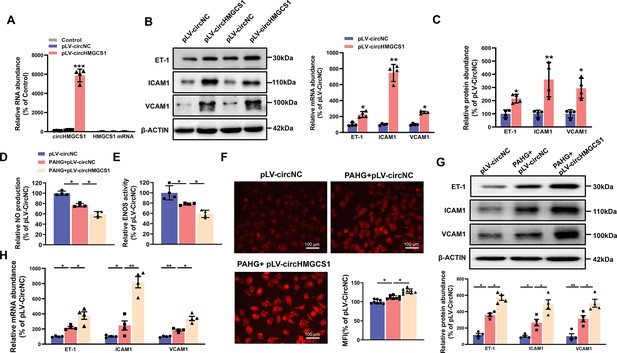
circHMGCS1 overexpression aggravates PAHG-induced endothelial dysfunction.
(A) HUVECs were transfected with either circHMGCS1 overexpression lentivirus (pLV-circHMGCS1) or lentiviral circular RNA negative control vector (pLV-circNC). After 48 hours of infection, circHMGCS1 and HMGCS1 expression levels were assessed by qRT-PCR and normalized to GAPDH (n=4). (B, C) Western blot and qRT-PCR were conducted to detect the expressions of adhesion molecules (VCAM1, ICAM1, and ET-1) in HUVECs between pLV-circHMGCS1 and pLV-circNC groups (n=4). (D) NO content in pLV-circHMGCS1-infected HUVECs after PAHG treatment (n=4). (E) ENOS activity in pLV-circHMGCS1-infused HUVECs after PAHG treatment (n=4). (F) DHE fluorescence was used to characterize ROS expression in different groups. Scale bar=100 μm (n=8). (G, H) Relative expression of adhesion molecules (ICAM1, VCAM1 and ET-1) in HUVECs infected with pLV-circNC or pLV-circHMGCS1 after PAHG treatment were determined by Western blot and qRT-PCR (n=4). *p<0.05, **p0.01, ***p<0.001, All significant difference was determined by one-way ANOVA followed by Bonferroni multiple comparison post hoc test, error bar indicates SD.
-
Figure 2—source data 1
Uncropped and labeled gels for (Figure 2).
- https://cdn.elifesciences.org/articles/97267/elife-97267-fig2-data1-v1.zip
-
Figure 2—source data 2
Raw unedited gels for (Figure 2).
- https://cdn.elifesciences.org/articles/97267/elife-97267-fig2-data2-v1.zip
MIR4521 protects endothelial cells from PAHG-induced dysfunction
circRNAs may function as ceRNAs to sponge miRNAs, thereby modulating miRNA target depression and imposing an additional level of post-transcriptional regulation in human diseases (Zhong et al., 2018). Accordingly, we conducted miRNA sequencing in PAHG-stimulated HUVECs to identify the potential miRNA targets of circHMGCS1. The deep sequencing analysis revealed 98 significantly differentially expressed miRNAs (Figure 3A and B, GEO submission: GSE237295). We then used the miRanda database and ceRNA theory to obtain four candidate miRNAs (MIR4521, MIR3143, MIR98-5P, and MIR181A-2–3 P; Figure 3C and D). qRT-PCR confirmed that all four miRNAs were downregulated (Figure 3E). Next, we observed that each of the four miRNA mimics induced a significantly increase in the expression of their corresponding miRNAs in HUVECs through the application of synthetic miRNA mimics (Figure 3—figure supplement 1A). Relative to the other three miRNAs, MIR4521 mimics significantly inhibited adhesion molecules (ICAM1, VCAM1, and ET-1) expression in HUVECs (Figure 3F and G), making it a suitable candidate for further endothelial function studies. We then investigate the effect of MIR4521 on PAHG-stimulated endothelial cell function using synthetic MIR4521 mimics and MIR4521 inhibitor in HUVECs (Figure 3—figure supplement 1B, C). Enhanced MIR4521 levels effectively restored PAHG-induced reductions in NO content and ENOS activity in HUEVCs, while MIR4521 inhibition led to opposite results (Figure 3H, I). whereas MIR4521 mimics significantly inhibited PAHG-induced expression of ROS and adhesion molecules (VCAM1, ICAM1, and ET-1; Figure 3J), whereas the MIR4521 inhibitor increased the PAHG-induced expression of ROS and adhesion molecules (VCAM1, ICAM1, and ET-1; Figure 3K and L and Figure 3—figure supplement 1D, E). These findings suggest that MIR4521 is involved in PAHG-induced endothelial cell dysfunction.
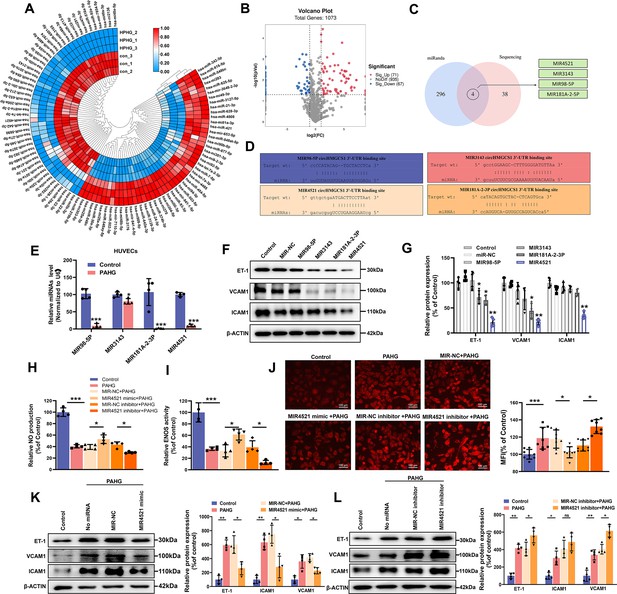
MIR4521 prevents PAHG-induced endothelial dysfunction.
(A) Heat map depicting differentially expressed miRNAs in HUVECs with or without PAHG treatment for 24 hr (n=3). (B) Volcano plot illustrating significant changes in miRNAs between control and PAHG-treated groups (Fold-change>2 or<0.5, p≤0.01). (C) Schematic illustration showing overlapping target miRNAs of circHMGCS1 predicated by miRanda and sequencing results. (D) The binding sites of circHMGCS1 were predicted using miRanda and involve MIR98-5P, MIR3143, MIR4521, and MIR181A-2–3 P. (E) Relative expression of four miRNA candidates in HUVECs treated with PAHG was assessed using qRT-PCR (n=4). (F, G) The regulatory effects of four miRNA mimics on the protein expression levels of adhesion molecules (ICAM1, VCAM1, and ET-1) (n=4). (H) Regulation of NO content by MIR4521 mimic or MIR4521 inhibitor under PAHG treatment (n=4). (I) Impact of MIR4521 mimic or MIR4521 inhibitor on ENOS activity during PAHG treatment. (n=4). (J) The ROS expression in MIR4521 mimic or MIR4521 inhibitor combined with PAHG treatment (Scale bar=100 µm, n=8). (K) Adhesion molecules (ICAM1, VCAM1, and ET-1) expression in PAHG-treated HUVECs transfected with MIR4521 mimics was determined by Western blot (n=4). (L) Determination of adhesion molecules (ICAM1, VCAM1, and ET-1) expression via Western blot in PAHG-treated HUVECs transfected with MIR4521 inhibitor (n=4). *p<0.05, **p<0.01, ***p<0.001, All significant difference was determined by one-way ANOVA followed by Bonferroni multiple comparison post hoc test, error bar indicates SD.
-
Figure 3—source data 1
Uncropped and labeled gels for (Figure 3).
- https://cdn.elifesciences.org/articles/97267/elife-97267-fig3-data1-v1.zip
-
Figure 3—source data 2
Raw unedited gels for (Figure 3).
- https://cdn.elifesciences.org/articles/97267/elife-97267-fig3-data2-v1.zip
circHMGCS1 acts as a MIR4521 sponge to regulate endothelial dysfunction
We then explored the interaction mechanism between circHMGCS1 and MIR4521. As displayed in Figure 4—figure supplement 1A, B, the overexpression of circHMGCS1 reduced the expression level of MIR4521, whereas knockdown of circHMGCS1 resulted in an upregulation of MIR4521. Meanwhile, bioinformatics analysis revealed the predicted binding sites between MIR4521 and circHMGCS1 (Figure 4—figure supplement 1C). We constructed a dual-luciferase reporter by inserting the wild-type (WT) or mutant (MUT) linear sequence of circHMGCS1 into the pmirGLO luciferase vector. The co-transfection of MIR4521 mimics with the circHMGCS1 luciferase reporter gene in HEK293T cells, reducing luciferase activity; mutations in the binding sites between circHMGCS1 and MIR4521 abrogated the effect of MIR4521 mimics on the activity of the circHMGCS1 luciferase reporter gene mutant (Figure 4A). Furthermore, AGO2 immunoprecipitation in HUVECs transfected with MIR4521 or its mutants demonstrated that MIR4521 facilitates the association of AGO2 with circHMGCS1 in HUVECs (Figure 4B). Meanwhile, we used biotinylated MIR4521 mimics in HUVECs stably overexpressing circHMGCS1 to further validate the direct binding of MIR4521 and circHMGCS1. The qRT-PCR results revealed that MIR4521 captured endogenous circHMGCS1, and the negative control with a disrupting putative binding sequence failed to coprecipitate circHMGCS1 (Figure 4C). Consistently, the use of biotin-labeled circHMGCS1 effectively captured both MIR4521 and AGO2 (Figure 4—figure supplement 1D, E). Moreover, the RNA-FISH assay showed colocalization of MIR4521 and circHMGCS1 in the cytoplasm (Figure 4D). Altogether, these findings indicate that circHMGCS1 functions as a molecular sponge for MIR4521.
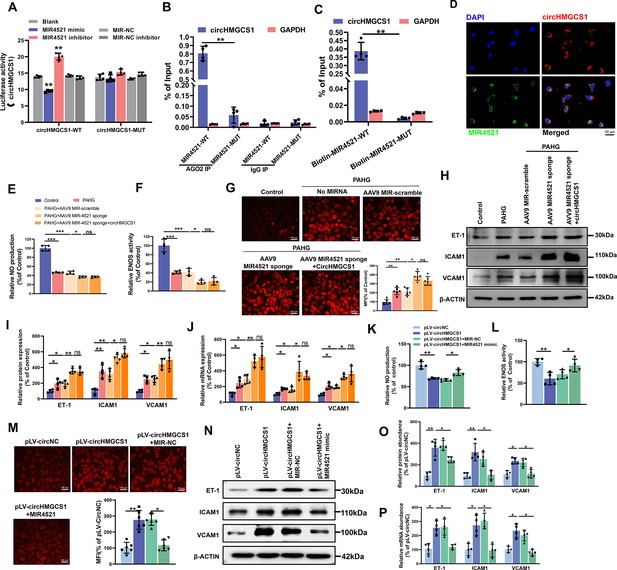
circHMGCS1 regulates PAHG-induced endothelial dysfunction by targeting and sponging MIR4521.
(A) Luciferase reporter constructs containing wild-type (WT) or mutant (MUT) circHMGCS1 were cotransfected with MIR4521 mimics, MIR-NC, MIR4521 inhibitor, or MIR-NC inhibitor in HEK293T cells (n=4). (B) Immunoprecipitation shows AGO2-mediated binding of circHMGCS1 and MIR4521 (n=4). (C) Biotin-coupled MIR4521 or its mutant probe was employed for circHMGCS1 pull-down, and captured circHMGCS1 level was quantified by qRT-PCR (n=4). (D) RNA-FISH showing the colocalization of circHMGCS1 and MIR4521 in HUVECs (red represents circHMGCS1, green represents MIR4521, blue represents nucleus, scale bar=50 μm, n=4). (E, F) circHMGCS1 exhibited no impact on NO content and ENOS activity in the presence of MIR4521 sponge (n=4). (G) circHMGCS1 demonstrated no influence on ROS expression with MIR4521 sponge (Scale bar=100 µm, n=8). (H–J) circHMGCS1 had no effect on the expression of adhesion molecules (ICAM1, VCAM1, and ET-1) in the presence of MIR4521 sponge which were determined by western blot and qRT-PCR (n=4). (K, L) MIR4521 attenuated the reduction of NO content and ENOS activity induced by circHMGCS1 (n=4). (M) MIR4521 inhibited the increase of ROS expression caused by circHMGCS1 (Scale bar=100 µm, n=8). (N–P) MIR4521 attenuated the increased expression of adhesion molecules (ICAM1, VCAM1, and ET-1) induced by circHMGCS1 which were determined by Western blot and qRT-PCR, respectively (n=4). *p<0.05, **p<0.01, ns means no significant. All significant difference was determined by one-way ANOVA followed by Bonferroni multiple comparison post hoc test, error bar indicates SD.
-
Figure 4—source data 1
Uncropped and labeled gels for (Figure 4).
- https://cdn.elifesciences.org/articles/97267/elife-97267-fig4-data1-v1.zip
-
Figure 4—source data 2
Raw unedited gels for (Figure 4).
- https://cdn.elifesciences.org/articles/97267/elife-97267-fig4-data2-v1.zip
Rescue experiments were conducted to investigate whether circHMGCS1 regulates endothelial cell function through MIR4521 sponging. We achieved MIR4521 inhibition by transfecting HUVECs with a recombinant adeno-associated virus 9 (AAV9) vector carrying MIR4521 sponges. Notably, MIR4521 sponges significantly promoted the PAHG-induced reduction in NO content and ENOS activity. Under this condition, increased circHMGCS1 expression did not interfere with the changes in NO content and ENOS activity (Figure 4E and F). Moreover, MIR4521 sponges elevated ROS expression in PAHG treated HUVECs, whereas no further additional effect on ROS expression was observed upon the overexpression of circHMGCS1 when MIR4521 was sponged (Figure 4G). Further evaluation of endothelial functional molecules revealed that circHMGCS1 failed to stimulate the expression of these factors when MIR4521 was sponged (Figure 4H–J). Furthermore, the reduction in NO content and ENOS activity induced by circHMGCS1 overexpression was reversed by MIR4521 mimics (Figure 4K and L), and the circHMGCS1-mediated increase in ROS content was inhibited by MIR4521 mimics (Figure 4M). Furthermore, the expression of adhesion molecules (ICAM1, VCAM1, and ET-1) was increased in circHMGCS1-transfected HUVECs, and this effect was significantly ameliorated by MIR4521 mimics (Figure 4N–P). These findings demonstrate that circHMGCS1 acts as a sponge for MIR4521, thereby regulating endothelial function.
circHMGCS1 serves as a MIR4521 sponge to regulate diabetes-induced VED
To further explore the interaction between MIR4521 and circHMGCS1 in diabetes-induced VED, we administered exogenous MIR4521 agomir (mimics) via tail vein injection to mice, whereas agomir NC, expressing random sequence, served as the negative control in HFHG-induced diabetes to examine its effect on diabetes and associated VED (Figure 5—figure supplement 1A). Compared with to non-diabetic WT (Control) mice, DM mice exhibited a significant increase in body weight, upon MIR4521 agomir application, the body weight of diabetic mice significantly decreased compared with the untreated diabetic mice (Figure 5A), and elevated fasting blood glucose levels in DM mice were likewise substantially inhibited (Figure 5B). By contrast, the combined treatment of circHMGCS1 and MIR4521 agomir did not significantly affect the body weight and blood glucose levels. OGTT and ITT experiments demonstrated that MIR4521 agomir considerably enhanced glucose tolerance and insulin resistance in diabetic mice (Figure 5C and D and Figure 5—figure supplement 1B, C). Blood lipid biochemistry analysis revealed that MIR4521 agomir restored abnormal blood lipid levels in diabetic mice (Figure 5—figure supplement 1D–G). Notably, the abundant presence of circHMGCS1 in the body can negate the inhibitory effect of MIR4521 on diabetes development. These results suggest that MIR4521 can inhibit the occurrence of diabetes, whereas circHMGCS1 specifically dampens the function of MIR4521, weakening its protective effect against diabetes.
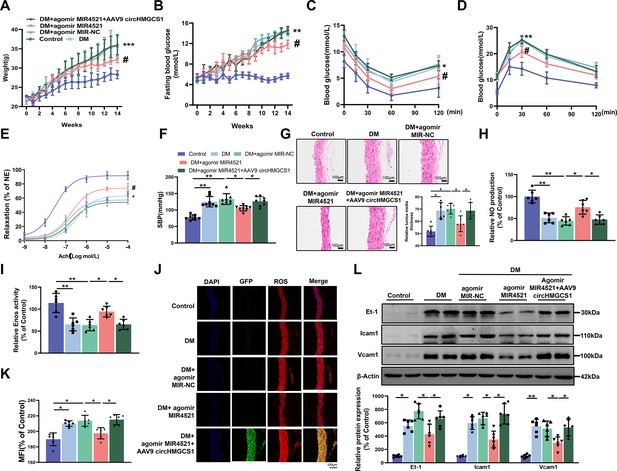
AAV9-mediated circHMGCS1 overexpression attenuates the protective effect of MIR4521 against diabetes-induced VED.
(A) Changes in body weight of mice from 0 to 14 weeks (n=8, ***p<0.001 DM versus control, #p<0.05 DM+agomir MIR4521 versus DM). (B) Alterations in fasting blood glucose in mice from 0 to 14 weeks (n=8, ***p<0.001 DM versus control, #p<0.05 DM+agomir MIR4521 versus DM). (C, D) ITT and OGTT were performed in the fasted mice (n=8, *p<0.05 DM versus control, #p<0.05 DM+agomir MIR4521 versus DM). (E) Relaxation responses of aortic rings from control, MIR4521 agomir, or MIR4521 agomir +AAV9 circHMGCS1 mice on DM diet (n=6). (F) SBP measured via tail-cuff method in different groups (n=8). (G) Hematoxylin and eosin staining (H&E) was performed on serial cross-sections of thoracic aortas from differently treated mice to assess vessel wall thickness. Scale bar=100 μm (n=6). (H, I) NO content and Enos activity in thoracic aorta after 6 weeks of DM diet with MIR4521 agomir or MIR4521 agomir +circHMGCS1 treatment (n=6). (J, K) Detection of ROS expression in thoracic aorta using DHE after MIR4521 agomir or MIR4521 agomir +AAV9 circHMGCS1 injection (red represents ROS, green represents GFP, blue represents nucleus, scale bar=100 μm, n=6). (L) Expression levels of adhesion molecules (Icam1, Vcam1, and Et-1) in thoracic aorta after 6 weeks of DM diet with MIR4521 agomir or MIR4521 agomir +circHMGCS1 treatment (n=6). *p<0.05, **p<0.01, ***p<0.001. All significant difference was determined by one-way ANOVA followed by Bonferroni multiple comparison post hoc test, error bar indicates SD.
-
Figure 5—source data 1
Uncropped and labeled gels for (Figure 5).
- https://cdn.elifesciences.org/articles/97267/elife-97267-fig5-data1-v1.zip
-
Figure 5—source data 2
Raw unedited gels for (Figure 5).
- https://cdn.elifesciences.org/articles/97267/elife-97267-fig5-data2-v1.zip
To investigate the roles of MIR4521 and circHMGCS1 in mouse vascular relaxation, we conducted an ex vivo culture of thoracic aortas from mice subjected to different treatments. A significant impairment in acetylcholine (Ach)-induced vascular relaxation response in the thoracic aorta of diabetic mice was markedly improved by MIR4521 agomir, emphasizing the critical involvement of MIR4521 in vascular relaxation. Conversely, circHMGCS1 inhibited the protective function of MIR4521 on vascular relaxation (Figure 5E). Across all experimental groups, the smooth muscle exhibited a normal endothelium-dependent relaxation response to the nitric oxide donor sodium nitroprusside (SNP; Figure 5—figure supplement 1H), revealing intact smooth muscle function. Meanwhile, diabetic mice displayed a significant increase in SBP compared with normal mice, which was significantly restrained by MIR4521 agomir treatment. Nevertheless, this beneficial effect was nullified in the presence of circHMGCS1 (Figure 5F). Furthermore, MIR4521 agomir inhibited intimal thickening and smooth muscle cell proliferation in diabetic mice, whereas upregulation of circHMGCS1 abrogated this effect (Figure 5G). These findings demonstrate that circHMGCS1 and MIR4521 play specific roles in regulating vascular endothelial function in diabetic mice.
We measured the NO level to further investigate the interaction between MIR4521 and circHMGCS1 in regulating diabetes-induced VED. Compared with the control group, the NO level decreased in the thoracic aorta of diabetic mice; however, MIR4521 agomir treatment increased its content (Figure 5H). Furthermore, MIR4521 treatment effectively enhanced Enos activity (Figure 5I), whereas circHMGCS1 hindered MIR4521 regulatory function on NO content and ENOS activity. Furthermore, MIR4521 reduced ROS production in the blood vessels (Figure 5J and K) and inhibited the expression of endothelial adhesion molecules (Icam1, Et-1, and Vcam1; Figure 5L). Nonetheless, in the presence of circHMGCS1, the effect of MIR4521 was abrogated. These results indicated that circHMGCS1 acts as a MIR4521 sponge, participating in diabetes-induced VED.
ARG1 is a direct target of MIR4521 to accelerate endothelial dysfunction
To investigate whether circHMGCS1-associated MIR4521 regulated VED-related gene expression by targeting the 3’-untranslated region (UTR), we conducted predictions of mRNAs containing MIR4521 binding sites using the GenCard, mirDIP, miRWalk, and Targetscan databases. Through bioinformatics analyses, we identified binding sites for MIR4521 on KLF6, ARG1, and MAPK1 genes (Figure 6A). Previous studies have demonstrated the involvement of elevated arginase activity in diabetes-induced VED. Arginase can modulate the production of NO synthesized by ENOS through competitive utilization of the shared substrate L-arginine (Jung et al., 2010; Romero et al., 2008). Moreover, MIR4521 possesses the capability to bind to the 3’ untranslated region of ARG1 in both human and mouse genomes (Figure 6—figure supplement 1A). The protein expression of Arg1 was increased in the aorta of diabetic mice and PAHG-treated HUVECs (Figure 6B and Figure 6—figure supplement 1B). The luciferase reporter assay was applied to verify the targeting ability through the pmirGLO vector, which included either the WT or MUT 3’-UTR of ARG1 (Figure 6—figure supplement 1C). The overexpression of MIR4521 reduced the luciferase activities of the WT reporter vector but not the MUT reporter vector (Figure 6C). We next investigated the function of MIR4521 and ARG1 to regulate the function of HUVECs. MIR4521 mimics recovered the reduced levels of NO and the compromised activity of ENOS triggered by ARG1 overexpression (Figure 6D and E and Figure 6—figure supplement 1D, E). Meanwhile, it inhibited the upregulation in ROS levels induced by ARG1 overexpression (Figure 6F and Figure 6—figure supplement 1F, G). The rescue experiment results demonstrated that MIR4521 mimics reversed the promotional effect of ARG1 on the mRNA and protein expression of adhesion molecules in vitro (Figure 6G and H). Subsequently, we evaluated the gene and protein expression levels of ARG1 using qRT-PCR and western blotting. MIR4521 mimics repressed ARG1 transcription and protein expression, whereas MIR4521 inhibition upregulated ARG1 expression (Figure 6I–K). Taken together, these results suggest that MIR4521 inhibits VED through sponging ARG1.
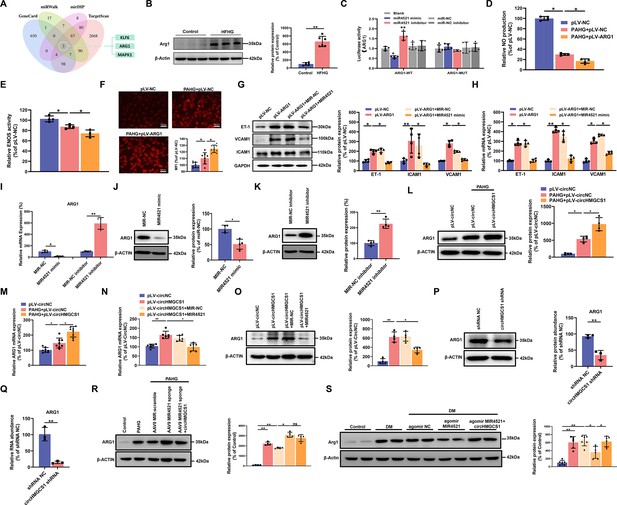
circHMGCS1 functions as a MIR4521 sponge in HUVECs to modulate ARG1 expression.
(A) Schematic depiction illustrating the intersection of predicted target genes of MIR4521 from TargetScan, miRWalk, mirDIP, and GeneCard. (B) Arg1 expression in thoracic aorta of DM by western blot (n=6). (C) HEK293T cells were cotransfected with MIR4521 mimics, MIR-NC, MIR4521 inhibitor, or MIR-NC inhibitor, along with luciferase reporter constructs containing WT or MUT 3′-untranslated region of ARG1 (n=4). (D, E) Modulatory effect of MIR4521 mimic on NO content and ENOS activity under ARG1 overexpression (n=4). (F) Regulatory impact of MIR4521 mimic on ROS content under ARG1 overexpression (Scale bar=100 µm, n=4). (G, H) ARG1-overexpressing HUVECs were generated using lentivirus and transfected with MIR4521 mimics for 24 hr to evaluate adhesion molecule expression (ICAM1, VCAM1, and ET-1) by western blot and qRT-PCR (n=4). (I–K) ARG1 expression was significantly decreased by MIR4521 mimics and increased by MIR4521 inhibitor, as determined by qRT-PCR and Western blot (n=4). (L, M) ARG1 expression was significantly increased by circHMGCS1 overexpression, as determined by western blot and qRT-PCR (n≥4). (N, O) circHMGCS1-overexpressed HUVECs, created with lentivirus and transfected with MIR4521 mimics for 48 hr, were examined for ARG1 expression by western blot and qRT-PCR (n=4). (P, Q) ARG1 expression was significantly reduced by circHMGCS1 shRNA, as determined by western blot and qRT-PCR (n=3). (R) AAV9 MIR4521 sponge was used to inhibit the MIR4521 expression in HUVECs, followed by circHMGCS1 transfection, and then treated with PAHG for 24 hr to evaluate the expression of ARG1 by western blot (n=4). (S) Detection of Arg1 expression in the thoracic aorta of DM treated with AAV9 MIR4521 agomir combined with AAV9 circHMGCS1 by western blot (n=4). *p<0.05, **p<0.01, ns means no significant. All significant difference was determined by one-way ANOVA followed by Bonferroni multiple comparison post hoc test, error bar indicates SD.
-
Figure 6—source data 1
Uncropped and labeled gels for (Figure 6).
- https://cdn.elifesciences.org/articles/97267/elife-97267-fig6-data1-v1.zip
-
Figure 6—source data 2
Raw unedited gels for (Figure 6).
- https://cdn.elifesciences.org/articles/97267/elife-97267-fig6-data2-v1.zip
To explore the regulatory effect of the interaction between circHMGCS1 and MIR4521 on ARG1 expression. we firstly used HUVECs employing overexpression of circHMGCS1. circHMGCS1 overexpression significantly further promoted ARG1 transcription and protein expression during the PAHG treatment (Figure 6L and M), confirming the regulatory function of circHMGCS1 in VED-related ARG1 expression. We next studied the function of MIR4521, which acts as a sponge for circHMGCS1 to regulate ARG1 in HUVECs. The mRNA and protein levels of ARG1 were significantly increased when HUVECs were transfected with circHMGCS1. However, MIR4521 overexpression counteracted the effects of circHMGCS1 on ARG1 mRNA and protein expression (Figure 6N and O). Conversely, knockdown of circHMGCS1 reduced ARG1 expression and increased MIR4521 expression in HUVECs (Figure 6P and Q and Figure 4—figure supplement 1B). Besides, the inhibition of MIR4521 using a MIR4521 sponge in HUVECs further increased ARG1 expression under the PAHG treatment. Notably, elevated circHMGCS1 expression did not affect ARG1 regulation (Figure 6R). Moreover, compared with diabetic mice, circHMGCS1 overexpression dampened the function of MIR4521 agomir on Arg1 (Figure 6S). These findings indicate that circHMGCS1 serves as a sponge for MIR4521, facilitating ARG1 regulation and VED promotion.
ARG1 is essential for circHMGCS1 and MIR4521 to regulate diabetes-induced VED
To delve further into how circHMGCS1 and MIR4521 regulated endothelial cell function via ARG1, we used AAV9 expressing ARG1 shRNA (ARG1 shRNA) or GFP (NC shRNA, used as the control) to reduce ARG1 levels. ARG1 shRNA mitigated the effects of PAHG on NO content and ENOS activity (Figure 7A and B), and inhibited PAHG-induced ROS in endothelial cells (Figure 7C). Additionally, ARG1 shRNA significantly reduced the elevated expression of adhesion molecules (ICAM1, VCAM1, and ET-1) induced by PAHG (Figure 7D and E). F Further rescue experiments revealed that MIR4521 and circHMGCS1 exhibited no significant regulatory effect on endothelial function in the presence of ARG1 shRNA. These findings demonstrate that ARG1 plays a crucial regulatory effect on MIR4521 and circHMGCS1 in modulating endothelial cell function.
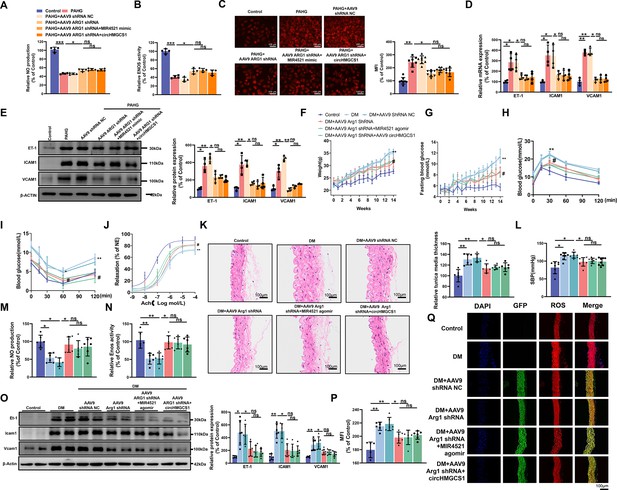
ARG1 is inseparable from circHMGCS1 and MIR4521 regulating diabetes-induced VED.
(A, B) circHMGCS1 and MIR4521 had no effect on NO content and ENOS activity expression in the absence of ARG1 (n=4). (C) ROS expression remained unaffected by circHMGCS1 and MIR4521 in the absence of ARG1 (n=4). (D, E) Relative expression of adhesion molecules (ICAM1, VCAM1, and ET-1) remained unchanged in the absence of ARG1, despite the presence of circHMGCS1 and MIR4521, as determined by qRT-PCR and Western blot (n=4). (F) Changes in mice body weight over the experimental period (n=8, **p<0.01, DM versus control, #p<0.05, DM +AAV9 ARG1 shRNA versus DM). (G) Fasting blood glucose levels in mice over time (n=8, **p<0.01, DM versus control, #p<0.05, DM +AAV9 ARG1 shRNA versus DM). (H, I) Blood glucose levels measured at week 13 (ITT) and week 14 (OGTT) (n=8, **p<0.01 DM versus control, #p<0.05, DM +AAV9 ARG1 shRNA versus DM). (J) Endothelium-dependent relaxations in aortic rings from different groups (n=8). (K) H&E performed on serial cross-sections of thoracic aortas from differently treated mice to evaluate vessel wall thickness. Scale bar=100 μm (n=6). (L) SBP measured by the tail-cuff method in different groups (n=8). (M, N) NO content and Enos activity expression in thoracic aort (n=6). (O) Relative expression of adhesion molecules (Icam1, Vcam1, and Et-1) in thoracic aorta assessed by Western blot (n=6). (P, Q) ROS expression in the thoracic aorta using the DHE probe (Red represents ROS, Green represents GFP, Blue represents nucleus, scale bar=100 μm, n=6). *p<0.05, **p<0.01, ns means no significant. All significant difference was determined by one-way ANOVA followed by Bonferroni multiple comparison post hoc test, error bar indicates SD.
-
Figure 7—source data 1
Uncropped and labeled gels for (Figure 7).
- https://cdn.elifesciences.org/articles/97267/elife-97267-fig7-data1-v1.zip
-
Figure 7—source data 2
Raw unedited gels for (Figure 7).
- https://cdn.elifesciences.org/articles/97267/elife-97267-fig7-data2-v1.zip
Considering the role of ARG1 in regulating endothelial cell function through circHMGCS1 and MIR4521, we downregulated ARG1 expression in mice by administering AAV9 ARG1 shRNA through tail vein injection (Figure 7—figure supplement 1A). As anticipated, AAV9 ARG1 shRNA-infused mice exhibited decreased body weight and lower fasting blood glucose levels compared with diabetic mice (Figure 7F and G). Moreover, OGTT and ITT demonstrated that reduced ARG1 levels in AAV9 ARG1 shRNA-treated mice preserved glucose tolerance and insulin sensitivity (Figure 7H, I and Figure 7—figure supplement 1B, C). The lowered ARG1 levels also reversed serum lipid levels (Figure 7—figure supplement 1D–G). However, the overexpression of circHMGCS1 or MIR4521 had no significant effect on glucose and lipid metabolism in AAV9 ARG1 shRNA-expressing mice. These findings confirm that ARG1 is an indispensable regulator for circHMGCS1 and MIR4521 in regulating diabetes.
We next investigated whether ARG1 regulates vascular endothelial cell function in the context of diabetes. Knocking down ARG1 in the thoracic aorta of mice expressing AAV9 ARG1 shRNA restored diastolic function of the thoracic aorta in diabetic mice (Figure 7J), emphasizing the vital role of ARG1 in VED. Furthermore, all experimental groups exhibited a normal endothelium-dependent relaxation response to the NO donor SNP, suggesting intact smooth muscle function (Figure 7—figure supplement 1H). Moreover, AAV9 ARG1 shRNA effectively inhibited diabetes-induced intima thickening and inhibited smooth muscle cell proliferation (Figure 7K), and which also counteracted the elevation of SBP induced by diabetes (Figure 7L). However, the regulatory effects of circHMGCS1 and MIR4521 on vascular dilation were ineffective in the presence of ARG1 shRNA. We next measured VED-related functional factors. Compared with diabetic mice, the thoracic aorta of mice expressing AAV9 ARG1 shRNA exhibited restored NO content and Enos activity (Figure 7M and N). Furthermore, knocking down ARG1 reduced the high expression levels of vascular endothelial adhesion molecules (Icam1, Et-1, and Vcam1) induced by diabetes (Figure 7O). Immunofluorescence analysis demonstrated a significant decrease in ROS content in the thoracic aorta of mice expressing AAV9 ARG1 shRNA compared with diabetic mice (Figure 7P and Q). Notably, circHMGCS1 and MIR4521 lost their regulatory capacity on vascular endothelial cell function in the presence of ARG1 shRNA. These results indicate that ARG1 plays a critical role in circHMGCS1 and MIR4521 to modulate diabetes-induced VED.
Discussion
The field of ncRNA biology has garnered considerable attention and has witnessed intensive research over the last few years. ncRNAs possess the capacity to function as novel regulators in various physiological systems and disease contexts (Esteller, 2011; Matsui and Corey, 2017; Statello et al., 2021). However, most studies have explored the expression patterns and functions of ncRNAs within single disease settings (Arcinas et al., 2019; Shan et al., 2017). The present study contributes to the understanding of ncRNAs and their involvement in diabetes-associated CVD, characterized by disruption of lipid metabolic homeostasis and redox balance in vascular endothelial cells under diabetic conditions. A comprehensive genome-wide analysis revealed 17,179 known circRNA loci transcribed in diabetic endothelial cells. Based on conservation, endothelial specificity, and abundance criteria, five circRNAs were selected for validation, indicating their predominant upregulation in the diabetic endothelial environment. Among them, we discovered a novel circRNA, circHMGCS1, exhibiting high abundance, stability, and cell specificity in diabetic endothelial cells and contributing to endothelial function regulation. Our findings establish that circHMGCS1 promotes VED progression, and its overexpression exacerbates endothelial cell dysfunction. Our study highlights the pivotal role of circHMGCS1 in regulating endothelial cell function during diabetes.
circRNAs play regulatory roles by acting as microRNA sponges or interacting with proteins to regulate alternative splicing, transcription, and epigenetic modifications (Arcinas et al., 2019; Hansen et al., 2013; Memczak et al., 2013). To investigate the mechanism of circHMGCS1 in VED regulation, we analyzed miRNA sequencing results and prediction software to identify MIR4521 as a novel endothelial-expressed miRNA. MIR4521 was found to possess a stable binding site with circHMGCS1, and its expression was significantly decreased in endothelial cells within a diabetic environment. Our functional studies revealed that MIR4521 mimics attenuated VED development in diabetes, whereas its inhibition exacerbated VED progression. These findings preliminarily demonstrate the regulatory ability of MIR4521 in VED. The interaction between circHMGCS1 and MIR4521 was further characterized through luciferase reporter gene assays, RNA pull-down, and RNA immunoprecipitation. AAV9-mediated circHMGCS1 overexpression in endothelial cells dampened MIR4521 expression and accelerated diabetes-induced VED. Conversely, restoring MIR4521 expression effectively alleviated VED progression, highlighting the critical role of MIR4521 in counteracting circHMGCS1-mediated promotion of diabetes-induced VED. Moreover, the absence of MIR4521 aggravated diabetes-induced VED, whereas exogenous circHMGCS1 addition did not affect VED development. Intriguingly, exogenous MIR4521 significantly restrained diabetes-induced VED exacerbation, which was attenuated upon the subsequent addition of exogenous circHMGCS1. These findings underscore the essential regulatory role of the circHMGCS1 and MIR4521 interaction in maintaining diabetic vascular homeostasis.
Finally, we investigated the downstream targets crucial for circHMGCS1-mediated endothelial cell function. Arginase is a dual-nucleus manganese metalloenzyme that catalyzes the hydrolysis of L-arginine, primarily producing L-ornithine and urea (Kanyo et al., 1996). ARG1 and ARG2—two isoforms of arginase—exist in vertebrates, including mammals. Although ARG1 and ARG2 share the same catalytic reaction, they are encoded by different genes and exhibit distinct immunological characteristics (Hara et al., 2020; Pudlo et al., 2017; Su et al., 2021). Elevated ARG1 activity in endothelial cells has been identified as a significant contributor to VED in T2DM individuals. This dysfunction is attributed to the excessive formation of ROS caused by the competition between ARG1 and ENOS for L-arginine. Consequently, oxidases or mitochondrial complexes become overactivated, resulting in reduced NO levels and subsequent VED (Caldwell et al., 2018). By contrast, inhibiting arginase has been shown to considerably enhance endothelial function in T2DM patients (Mahdi et al., 2018; Shemyakin et al., 2012; Zhou et al., 2018). Our findings suggest that T2DM enhances ARG1 activity and expression in vascular endothelial cells. Conversely, ARG1 inhibition in endothelial cells delayed the onset of diabetes and preserved NO metabolite levels in the aorta. Additionally, Ach-induced vasodilation remains intact in the absence of ARG1 in the aortic ring, as ARG1 inhibition promotes ENOS-dependent relaxation, thereby preventing VED. Meanwhile, ROS content in the aorta of diabetic mice could be reduced under ARG1 inhibition, thereby suppressing the occurrence of oxidative stress. AAV9-mediated overexpression of circHMGCS1 increases ARG1 expression, which is inhibited by exogenous MIR4521 intervention. In the absence of MIR4521, circHMGCS1 cannot regulate ARG1 expression, whereas exogenous MIR4521 intervention inhibits the diabetes-induced increase in ARG1 expression in vivo, and this effect is counteracted by circHMGCS1 overexpression. Both in vitro and in vivo data suggest that the MIR4521 or circHMGCS1 fails to regulate the effect of diabetes-induced VED in the absence of ARG1. Therefore, ARG1 may serve as a promising VED biomarker, and circHMGCS1 and MIR4521 play a key role in regulating diabetes-induced VED by ARG1.
It would be intriguing to elucidate the mechanisms through which circHMGCS1 and MIR4521 exert their regulatory roles in endothelial cell function. The present study elucidated the potential VED-associated mechanisms in diabetes, with a specific focus on the complicated mutual effects between circHMGCS1 and MIR4521. The ceRNA network involving circHMGCS1-MIR4521-ARG1 can become a novel and significant regulatory pathway for preventing and potentially treating diabetes-induced VED. Our findings suggest that targeted inhibition of circHMGCS1 or the overexpression of MIR4521 could serve as effective strategies in mitigating diabetes-induced VED. These insights underscore the promising function of ncRNAs in developing therapeutic interventions for diabetes-associated VED.
Materials and methods
Reagent type (species) or resource | Designation | Source or reference | Identifiers | Additional information |
---|---|---|---|---|
Cell line (Homo sapiens) | HUVEC | The Shanghai Cell Bank of the Chinese Academy of Sciences | RRID:CVCL_2959 | |
Cell line (H. sapiens) | HEK-293T | The Shanghai Cell Bank of the Chinese Academy of Sciences | RRID:CVCL_0063 | |
Gene (H. sapiens) | circHMGCS1 | circbase | circRNA ID: hsa_circ_0008621 | |
Gene (H. sapiens) | HMGCS1 | GeneBank | Gene ID: 3157 | |
Gene (H. sapiens) | MIR4521 | miRbase | miRNA ID: MIMAT0019058 | |
Gene (Mus musculus) | ARG1 | GeneBank | Gene ID: 383 | |
Gene (M. musculus) | Arg1 | GeneBank | Gene ID: 11846 | |
Antibody | Anti- Liver Arginase (ARG1) (Rabbit monoclonal) | Abcam | ab133543 | WB (1: 1000) |
Antibody | Anti- Arg2 (Rabbit monoclonal) | Abcam | ab264066 | WB (1: 1000) |
Antibody | Anti- Endothelin 1(ET-1)(Mouse monoclonal) | Abcam | ab2786 | WB (1: 1000) |
Antibody | Anti- VCAM1 (Rabbit monoclonal) | Abcam | ab134047 | WB (1: 1000) |
Antibody | Anti-ICAM1 (Rabbit monoclonal) | Abcam | ab222736 | WB (1: 1000) |
Antibody | Anti-AGO2 (Mouse monoclonal) | Proteintech | 67934–1-Ig | WB (1: 2000) |
Antibody | Anti-beta ACTIN (Mouse monoclonal) | Abcam | ab8226 | WB (1: 2000) |
Antibody | Anti-GAPDH (Mouse monoclonal) | Abcam | ab8245 | WB (1: 2000) |
Recombinant DNA reagent | pCDH-CMV-MCS-EF1-Puro (plasmid) | Addgene | RRID:Addgene_73030 | |
Recombinant DNA reagent | pLKO.1-GFP-shRNA (plasmid) | Addgene | RRID:Addgene_30323 | |
Recombinant DNA reagent | psPAX2(plasmid) | Addgene | RRID:Addgene_12260 | |
Recombinant DNA reagent | pMD2G(plasmid) | Addgene | RRID:Addgene_12259 | |
Recombinant DNA reagent | pAAV-MCS (plasmid) | Ruipute | Cat#: 2212E4 | |
Recombinant DNA reagent | pAAV-RC9 (plasmid) | Ruipute | Cat#: 23011 | |
Recombinant DNA reagent | pHelper (plasmid) | Ruipute | Cat#: 230112 | |
Recombinant DNA reagent | pAAV-MIR4521 sponge-zsGREEn1-shRNA(plasmid) | Ruipute | Cat#: 230113 | |
Recombinant DNA reagent | pAAV-Arg1-zsGREEn1-shRNA(plasmid) | Ruipute | Cat#: 2212E1 | |
Recombinant DNA reagent | pAAV-ARG1-zsGREEn1-shRNA(plasmid) | Ruipute | Cat#: 2212E2 | |
Recombinant DNA reagent | pAAV-ZsGreen1 (plasmid) | Takara | Cat#: 6231 | |
Recombinant DNA reagent | pAAV-ZsGreen1-shRNA (plasmid) | youbio | Cat#: VT8093 | |
Commercial assay or kit | Fluorescent In Situ Hybridization Kit | RIBOBIO | Cat#: C10910 | |
Commercial assay or kit | RNA pull down kit | GENESEED | Cat#: P0202 | |
Commercial assay or kit | RNA Immunoprecipitation Kit | GENESEED | Cat#: P0102 | |
Software, algorithm | ImageJ | National Institutes of Health | https://imagej.nih.gov/ij/ | |
Software, algorithm | Prism | GraphPad v8.0 | RRID:SCR_002798 |
Cell culture
Request a detailed protocolHUVECs were cultured in high glucose Dulbecco’s Modified Eagle Medium (DMEM; Hyclone, USA) supplemented with 10% fetal bovine serum (FBS; Gibco, USA), 100 μg/mL of streptomycin, and 100 U/mL of penicillin. The cells were cultured at 37 °C in a humidified incubator with 95% air and 5% CO2. To simulate the elevated glucose levels observed in diabetes, we cultured HUVECs in a PAHG medium. The medium contained 25 mM glucose and 250 μM saturated free fatty acid (FFA) palmitate (16:C; Sigma, USA) and was incubated for 24 hr. For in vitro experiments, HUVECs were subcultured in six-well plates. The cells were then transfected with MIR4521 mimics, MIR-inhibitor, or MIR-NC and incubated for 48 hr. Subsequently, the cells were treated with PAHG for another 24 hr. Samples were collected to determine the corresponding indices.
Animal design
Request a detailed protocolMale C57BL/6 J mice (6–8 weeks old) were obtained from SLAC Laboratory Animal Co., Ltd. (SLAC ANIMAL, China). The mice were housed at a temperature of 22 ± 1 °C with a 12 hr light/dark cycle. To induce diet-induced diabetes, we fed wild type littermates either a standard chow (Control) or a high fat-high sucrose (HFHG) diet, where the diet composition consisted of 60% fat, 20% protein, and 20% carbohydrate (H10060, Hfkbio, China). The dietary regimen was maintained for 14 weeks. Throughout this period, body weight and fasting blood glucose (FBG) levels were measured on a weekly basis. An FBG level of ≥11.1 mmol/L was used as the criterion for a successful diabetic model. To study the role of ARG1 in diabetes-induced endothelial dysfunction, we established an HFHG-induced diabetic model (DM), and then pAAV9-Arg1 shRNA (1×1012 vg/mL, 100 µL) was injected into the tail vein. Subsequently, we observed changes in relevant indicators. To investigate the regulatory relationship among Arg1, MIR4521, and circHMGCS1 in diabetes-induced endothelial dysfunction, we injected MIR4521 agomir or pAAV9-circHMGCS1 via the tail vein, following the procedure described in Figure 5—figure supplement 1A. The mice were sacrificed after a 12 hr fast, and blood and other tissues were collected and stored at –80 °C until further analysis. All animal experiments were conducted in strict accordance with the guidelines and laws governing the use and care of laboratory animals in China (GB/T 35892–2018 and GB/T 35823–2018) and NIH Guide for the Care and Use of Laboratory Animals. The experimental procedures involving animals were performed at the Animal Experiment Center of Zhejiang Chinese Medical University in Hangzhou, China. The animal protocol was conducted in compliance with the guidelines set forth by the Ethics Committee for Laboratory Animal Care at Zhejiang Chinese Medical University (Approval No. 2022101345).
Vascular function
Request a detailed protocolAfter sacrificing the mice, the thoracic aortae and mesenteric arteries were carefully removed and dissected in an oxygenated ice-cold Krebs solution. The changes in the isometric tone of the aortic rings and second-order mesenteric arteries were recorded using a wire myograph (Danish Myo, DK). The arterial segments were stretched to achieve an optimal baseline tension (3 mN for the aorta) and allowed to equilibrate for 1 hr. Subsequently, the segments were contracted using 60 mmol/L of KCl and rinsed with Krebs solution. Endothelium-dependent relaxation (EDR) was evaluated by assessing the concentration-responses to cumulative additions of acetylcholine (ACh) in noradrenaline (NE, 10–3 mol/L) precontracted rings.
Blood pressure measurements
Request a detailed protocolAn intelligent non-invasive blood pressure monitor (BP-2010AUL) was used to measure blood pressure in different mouse groups simultaneously. Prior to measurement, the mice were placed in a blood pressure room until reaching a temperature of 25 ± 1 °C. After a 15 min equilibration period, the mice were gently positioned in a mice jacket set at a constant temperature of 40 °C, with their tail inserted into a pulse sensor. Throughout the measurements, a quiet environment was maintained to minimize external disturbances. The instrument automatically recorded the stabilized sensor signal to obtain the systolic blood pressure (SBP).
Serum lipid assays
Request a detailed protocolAfter 5 weeks of blood collection from mouse orbits and 12 weeks of drug administration, blood samples were obtained from mouse arteries. The collected blood was centrifuged at 1500 × g for 15 min to separate the serum. Commercial kits and an automatic biochemical analyzer (Biobase BK-600) were used to measure triglycerides (TG), total cholesterol (TC), high-density lipoprotein cholesterol (HDL-c), and low-density lipoprotein cholesterol (LDL-c).
Construction of circHMGCS1 and ARG1 plasmids and stable transfection using lentivirus circHMGCS1-overexpressing lentiviruses (pLV-circHMGCS1) were obtained from Hangzhou Ruipu Biotechnology Co., Ltd (Guangzhou, China). The circHMGCS1 sequence [NM_001098272: 43292575–43297268], the splice site AG/GT and ALU elements were inserted into the pCDH-circRNA-GFP vector (upstream ALU: AAAGTGCTGAGATTACAGGCGTGAGCCACCACCCCCGGCCCACTTTTTGTAAAGGTACGTACTAATGACTTTTTTTTTATACTTCAG, downstream ALU: GTAAGAAGCAAGGAAAAGAATTAGGCTCGGCACGGTAGCTCACACCTGTAATCCCAGCA). The restriction enzyme sites selected were EcoRI and NotI. Lentiviruses containing an empty vector and expressing GFP were used as the negative control (pLV-NC; Liang and Wilusz, 2014). ARG1-overexpressing lentiviruses (pLV-ARG1 OE) were acquired from Genomeditech (China). HUVECs were cultured in six-well plates until reaching 60% confluence and then infected with lentivirus particles in the presence of 5 μg/ml of polybrene at a multiplicity of infection of 30. The cells were cultured for at least 3 days before further experiments were conducted. The overexpression efficiency of circHMGCS1 or ARG1 was evaluated using quantitative real-time PCR or western blottings.
Cloning and production of circHMGCS1, MIR4521 sponge, circHMGCS1 shRNA and ARG1 shRNA
Request a detailed protocolThe pAAV-circHMGCS1-ZsGreen1 vector was constructed by inserting the full-length circHMGCS1 into the pAAV-circRNA-ZsGreen1 vector. circHMGCS1 shRNA vector was constructed by inserting circHMGCS1 shRNA (5'-ACAUAGCAACUGAGGGCUUCG-3') into pLKO.1 GFP shRNA plasmid. To achieve AAV-mediated ARG1 gene silencing, we obtained a shRNA sequence targeting ARG1 from Sigma-Aldrich Mission RNAi (TRCN0000101796). The oligo sequences 5’GATCCGCCTTTGTTGATGTCCCTAATCTCGAGATTAGGGACATCAACAAAGGCTTTTTA-3’ and 5’-AGCTTAAAAAGCCTTTGTTGATGTCCCTAATCTCGAGATTAGGGACATCAACAAAGGCG-3’ were synthesized, annealed, and ligated to the pAAV-ZsGreen-shRNA shuttle vector, resulting in the construction of pAAV-shARG1-ZsGreen1. The MIR4521 sponge cassette was cloned into the pAAV-ZsGreen1 expression vector to generate pAAV-MIR4521 sponge-ZsGreen1. Recombinant AAVs (rAAV9s) were produced by transfecting HEK293T cells plated at a density of 1×107 cells/15 cm plate 1 day prior to transfection using polyethyleneimine (Linear PEI, MW 25 kDa, Sigma-Aldrich) as the transfection reagent. For each 15 cm plate, the following reagents were added: 20 μg of pHelper, 10 μg of pAAV-RC9, and 10 μg of pAAV-circHMGCS1-ZsGreen1, pAAV-shARG1-ZsGreen1, or pAAV-MIR4521 sponge-ZsGreen1. These plasmids were combined with 500 μL of serum-free and antibiotic-free DMEM and 100 μL of PEI reagent (1 mg/mL, pH 5.0). The DNA-PEI reagent was added drop-wise to the cells without changing the media on the 15 cm plates. After three days, the cells were collected and lysed through repeated cycles of freezing and thawing at −80 °C and 37 °C, respectively. The rAAV9s were purified using the ViraTrap Adenovirus Purification Maxiprep Kit (BW-V1260-02, Biomiga). The virus titer was determined using qPCR and adjusted to 1×1012 viral genomes (vg)/mL in PBS containing 4% sucrose.
Transfection of miRNA mimic or inhibitor
Request a detailed protocolMIR4521 mimics, miRNA negative control (MIR-NC), MIR4521 inhibitor, and miRNA inhibitor negative control (MIR-NC inhibitor) were obtained from Tingske (China). To investigate the functional role of MIR4521 in HUVECs, we performed transfections using Hieff Trans in vitro siRNA/miRNA Transfection Reagent with MIR4521 mimics, MIR-NC, MIR-NC inhibitor, or MIR-NC inhibitor at a concentration of 50 nM, following the manufacturer’s protocols. After 48 hr of transfection, proteins and RNA were collected for further analysis. The sequences used in the experiments are listed as follows: miRNA negative control (MIR-NC): 5’-UUCUCCGAACGUGUCACGUTT-3’, 5’-ACGUGACACGUUCGGAGAATT-3’;MIR98-5P-mimics: 5’-UGAGGUAGUAAGUUGUAUUGUU-3’, 5’-CAAUACAACUUACUACCUCAUU-3’; MIR3143-mimics: 5’-UAACAUUGUAAAGCGCUUCUUU-3’, 5’-AGAAGCGCUUUACAAUGUUAUU-3’; MIR181A-2–3 P-mimics: 5’-ACCACUGACCGUUGACUGUACC-3’, 5’-UACAGUCAACGGUCAGUGGUUU-3’; MIR4521-mimics: 5’-GAGCACAGGACUUCCUUAGCUU-3’, 5’-GCUAAGGAAGUCCUGUGCUCAG-3’; MIR4521-inhibitor: 5’-CUGAGCACAGGACUUCCUUAGC-3’; miRNA inhibitor negative control (MIR-NC inhibitor): 5’-CAGUACUUUUGUGUAGUACAA-3’; MIR4521 agomir: 5’-GAGCACAGGACUUCCUUAGCUU: 5’-GCUAAGGAAGUCCUGUGCUCAG-3’.
NO assays
Request a detailed protocolThe supernatant fluid from HUVECs treated with the indicated reagents and a tissue homogenate of aortic rings were collected. NO concentrations were determined using the Griess reagent and a total nitric oxide assay kit (Beyotime, China) following the manufacturer’s instructions.
ENOS activity assay
Request a detailed protocolThe cellular ENOS activity was assessed as per previously described protocol. (Leopold et al., 2007). Briefly, Cells were exposed to a PBS buffer (400 μL/well) containing 1.5 Ci/ml [3 H] L-arginine for 15 min to initiate ENOS activity measurement. The reaction was stopped using 1 N ice-cold TCA (500 μL/well). After freeze-thawing in liquid nitrogen, cells were scraped, treated with water-saturated ether three times, and neutralized with 1.5 ml of 25 mM pH 8.0 HEPES. Dowex AG50WX8 columns (Tris form) were used for elution with a 1 ml solution of 40 mM pH 5.5 HEPES supplemented with 2 mM EDTA and 2 mM EGTA. The eluate was collected for [3 H] L-citrulline quantification using liquid scintillation spectroscopy. Additionally, the assessment of ENOS activity in cell lysates and tissues was performed through the previously outlined immunoprecipitation approach (Du et al., 2001). Briefly, the samples were divided into two tubes: one for protein blotting and the other for ENOS activity measurement. The ENOS immunocomplexes, linked to protein A-Sepharose beads, were reconstituted in assay buffer, and ENOS activity was gauged by tracking the transformation of [3 H] L-arginine into [3 H] L-citrulline. Equal enzyme quantities in each incubation were validated via western blotting.
Detection of ROS for tissue and cell
Request a detailed protocolTo assess the level of ROS in tissues, we used a red fluorescent reactive oxygen probe called DHE (dihydroethidium; Karim et al., 2022; Su et al., 2018). In brief, 10 μL of the DHE probe was combined with 190 μL of tissue homogenate supernatant, thoroughly mixed, and subsequently incubated in darkness at 37 °C for 30 min. Following incubation, the samples were promptly imaged under a fluorescent microscope.
For ROS detection in cells, the treated cells were washed once by PBS, then 20 μM DHE was added, and incubated at 37 °C for 30 min away from light, then washed three times by PBS and then colorless DMEM medium was added, followed by fluorescence microscopy for observation.
CircRNA sequencing
Request a detailed protocolTotal RNA was extracted from HUVECs, both with and without PAHG stimulation, utilizing Trizol (Invitrogen, Carlsbad, USA) following the manufacturer’s instructions. The extracted total RNA was then assessed for quality and quantity using a denaturing agarose gel, Nano Drop, and Agilent 2100 bioanalyzer (Thermo Fisher Scientific, USA). For the construction of RNA-seq libraries to obtain sequence information of linear transcripts, 3 μg of total RNA samples underwent treatment with the epicenter Ribo-ZeroTM Kit (Illumina, San Diego, USA) to remove rRNA. Linear RNA was subsequently eliminated using RNase R (Epicentre Technologies, USA). The resulting cleaved RNA fragments were reverse-transcribed to generate cDNA, which served as the template for the synthesis of U-labeled second-stranded DNAs. This synthesis step involved the use of E. coli DNA polymerase I, RNase H, and dUTP, facilitated by the PrimeScript RT regent Kit (TaKaRa, Japan). Purification of the synthesized second-stranded DNAs was accomplished using AMPureXP beads. All subsequent steps were conducted in accordance with the manufacturer’s protocols. To assess the quality of the libraries, an Agilent 2100 Bioanalyzer was employed, and the paired-end sequencing was performed using an Illumina Hiseq 4000 (LC Bio, China), adhering to the recommended protocol provided by the vendor.
Treatment of circRNA raw sequencing data
Request a detailed protocolThe sequencing data was filtered with Cutadapt by removing reads containing adaptor contamination, poly-N or low quality and undetermined bases, and then sequence quality was verified using FastQC (http://www.bioinformatics.babraham.ac.uk/projects/fastqc/) (Kechin et al., 2017). Bowtie2 and Hisat2 to map reads to the genome of species (Kim et al., 2015; Langmead and Salzberg, 2012). CIRCExplorer2 and CIRI were used to de novo assemble the mapped reads to circular RNAs (Kim and Salzberg, 2011; Zhang et al., 2016). Then, back splicing reads were identified in unmapped reads by tophat-fusion. All samples were generated unique circular RNAs. The expression level was calculated according to the junction reads per billion mapped reads (RPB). Differentially expressed circRNAs analysis was performed with log2 (fold change)>1 or log2 (fold change) <-1 and with statistical significance (p-value <0.05) by R package–edgeR (Robinson et al., 2010).
microRNA microarray assay
Request a detailed protocolTotal RNA was extracted from HUVECs treated with either PAHG or PBS using TRIzol reagent (Invitrogen, USA). The μParaflo MicroRNA microarray Assay by LC Sciences (LC Sciences, USA) using miRBase version 22.0 was performed. After 3'-extension with a poly(A) tail and ligating an oligonucleotide tag, hybridization was done overnight on a μParaflo microfluidic chip. Cy3 dye facilitated dye staining after RNA hybridization. Fluorescence images were acquired using the GenePix 400B laser scanner (Molecular Devices, USA). Data were analyzed by background subtraction and signal normalization using a LOWESS filter. miRNA expression with a two-tailed p-value of <0.05 was considered significant. Intensities below 500 were considered false positives.
Network analysis of competing endogenous RNA
Request a detailed protocolThe miRNA targets of circHMGCS1 were computationally predicted using three bioinformatic tools: miRanda (http://www.microrna.org/microrna/home.do), whereas the potential target genes of MIR4521 were envisaged using four distinct databases: TargetScan (http://www.targetscan.org/), GenCard (https://www.genecards.org/), miRWalk (http://mirwalk.umm.uni-heidelberg.de/), and mirDIP (http://ophid.utoronto.ca/mirDIP/).
Isolation of RNA and miRNA for quantitative real time-PCR (qRT-PCR) analysis
Request a detailed protocolRNA was extracted utilizing a total RNA extraction kit (Yeason, 19221ES50, China) and a miRNA extraction kit (Yeason, 19331ES50, China) according to the corresponding manufacturer’s protocol. The RNA concentration was determined at 260 nm using a spectrophotometer. Reverse transcription was conducted using a reverse transcription kit (TAKARA, Japan) with random primers according to the manufacturer’s protocol to synthesize cDNA and circRNA. Quantitative real-time PCRs (qRT-PCRs) were conducted on the CFX96 Touch Real-Time PCR Detection System (Bio-Rad, USA) using the standard protocol provided by the SYBR Green PCR Kit (Yeason, China). For miRNA expression detection, reverse transcription was executed, and microRNAs were detected using stem-loop primers procured from Tsingke (China). The relative gene expression was calculated using the 2-ΔΔCt method. All qRT-PCR assays were repeated thrice. The primers used for the qRT-PCR assays are listed as follows: MIR98-5P: 5’-CGCGCGTGAGGTAGTAAGTTGT-3’, 5’-AGTGCAGGGTCCGAGGTATT-3’; MIR3143: 5’-GCGATAACATTGTAAAGCGCTT-3’, 5’-AGTGCAGGGTCCGAGGTATT-3’; MIR181A-2–3 P: 5’-GCGACCACTGACCGTTGAC-3’, 5’-AGTGCAGGGTCCGAGGTATT-3’; MIR4521: 5’-CGGCTAAGGAAGTCCTGTGC-3’, 5’-CGCAGGGTCCGAGGTATTC-3’; U6: 5’-ATTGGAACGATACAGAGAAGATT-3’, 5’-GGAACGCTTCACGAATTTG-3’; ET-1: 5’-GCCTGCCTTTTCTCCCCGTTAAA-3’, 5’-CAAGCCACAAACAGCAGAGA-3’; ICAM1: 5’-TGACCGTGAATGTGCTCTCC-3’, 5’-TCCCTTTTTGGGCCTGTTGT-3’; ARG1: 5’-TTCTCAAAGGGACAGCCACG-3’, 5’-CGCTTGCTTTTCCCACAGAC-3’; ICAM1: 5’-ATGCCCAGACATCTGTGTCC-3’, 5’-GGGGTCTCTATGCCCAACAA-3’; VCAM1: 5’-AAATCGAGACCACCCCAGAA-3’, 5’-AGGAAAAGAGCCTGTGGTGC-3’; β-ACTIN: 5’-CTCACCATGGATGATGATATCGC-3’, 5’-CACATAGGAATCCTTCTGACCCA-3’; HMGCS1: 5’-TCGTGGGACACATATGCAAC-3’, 5’-TGGGCATGGATCTTTTTGCAG-3’; hsa_circ_0000992: 5’-TCACACGGGAGAGTGTTACC-3’, 5’-GTCCATCGAGAAAAGCTGATGC-3’; hsa_circ_0004036: 5’-GGCTCACAAGCAGCCTTTAC-3’, 5’-GGTGATACAGGAGCGGGTAG-3’; hsa_circ_0004889: 5’-GTCATATATGGGAAAGAGGGCTT-3’, 5’-GGACAAGCCTTCATGGGCTC-3’; hsa_circ_0006719: 5’-CCTCCCTCGGTGTTGCCTTC-3’, 5’-TCCAGGCCAGGTAGACAGAAC-3’; hsa_circ_0008621: 5’-CAAACATAGCAACTGAGGGCTTC-3’, 5’-TAATGCACTGAGGTAGCACTG-3’; GAPDH: 5’-GAAAGCCTGCCGGTGACTAA-3’, 5’-GCATCACCCGGAGGAGAAAT-3’.
circRNA validation by PCR
Request a detailed protocolDNA and gDNA templates were PCR amplified using BioRad Mastercyclers following the manufacturer’s protocol. Subsequently, the PCR products were visualized using a 1% agarose gel stained with GelRed. To validate the PCR results, we purified the products using the EZ-10 Column DNA Purification Kit (Sangon Biotech, China), and direct PCR product Sanger sequencing was performed. circHMGCS1-full length: 5′-AGCAACTGAGGGCTTCGT-3′,5′-ATGTTTGAATGCACAAGTCCTAC-3′;circHMGCS1-divergent primers: 5′-TTATGGTTCTGGTTTGGCTGC-3′, 5′-TTCAGCGAAGACATCTGGTGC-3′; circHMGCS1-convergent primers: 5′-ATAGCAACTGAGGGCTTCGTG-3′, 5′-GCGGTCTAATGCACTGAGGT-3′.
Cell nuclear/cytoplasmic fractionation
Request a detailed protocolRNA extracts from HUVECs were subjected to nuclear/cytoplasmic isolation using the NE-PER Nuclear and Cytoplasmic Extraction Reagent (Thermo Fisher Scientific, USA) following the manufacturer’s protocol.
RNase R treatment
Request a detailed protocolFor RNase R treatment, approximately 2 μg of total RNAs from HUVECs were incubated with or without 20 U of RNase R (Geneseed, China) at 37 °C for 30 min. The resulting RNAs were then purified using an RNA Purification Kit (QIAGEN, USA).
Actinomycin D assay
Request a detailed protocolHUVECs were seeded equally in 24-well plates at a density of 5×104 cells per well. Subsequently, the cells were exposed to actinomycin D (2 µg/mL; Abcam, USA) for different time intervals: 0, 4, 8, 12, and 24 hr. After the respective exposure times, the cells were collected for RNA extraction. The relative RNA levels of circHMGCS1 and HMGCS1 mRNA were analyzed using qRT-PCR and normalized to the values obtained from the control group (0 hr).
RNA immunoprecipitation
Request a detailed protocolRIP assay was conducted using the PureBindingRNA Immunoprecipitation Kit (Geneseed, China) as per the manufacturer’s guidelines. HUVECs were lysed in complete RNA immunoprecipitation lysis buffer containing 1% proteinase inhibitor and 1% RNase inhibitor after being infected with circNC or circHMGCS1. The cell extract was then incubated with magnetic beads conjugated with anti-Argonaute 2 (AGO2) or anti-IgG antibody (Abcam, USA) overnight at 4 °C. The beads were subsequently washed, and the proteins were removed using columns. Eventually, the isolated RNA was extracted using TRIzol Reagent, and the purified RNA was utilized for subsequent qRT-PCR analysis.
Pull-down assay
Request a detailed protocolA pull-down assay was conducted using the PureBinding RNA-Protein pull-down Kit (Geneseed, P0201, China) following the manufacturer’s protocol. The pull-down assay with biotinylated MIR4521 or circHMGCS1 was performed as previously described (Wang et al., 2020). Briefly, HUVECs were transfected with biotinylated RNA or mutants (Tingke, 50 nmol/L) using the Hieff Trans in vitro siRNA/miRNA Transfection Reagent (Yeason, China). Following a 48 hr transfection period, the cells were collected, PBS-washed, and incubated for 10 min in a capture buffer on ice. A 10% fraction of the cell lysates was retained as input. The remaining lysates were subsequently exposed to streptavidin magnetic beads for 30 min at 4 °C. After treatment with wash buffer and the RNeasy Mini Kit (QIAGEN), the captured RNAs were extracted for subsequent qRT-PCR analysis,while the associated proteins were processed for western blot analysis.
The sequences are listed as follows: Biotin-MIR4521-MUT: 5’-GCAUUCCUUCAGGAGUGCUCAG-3’, Biotin-MIR4521-WT: 5’-GCUAAGGAAGUCCUGUGCUCAG-3’. Biotin-circHMGCS1-WT: 5’- ATGTGTCCCACGAAGCCCTCAGTTGCTATGTTTGAA-3’, Biotin-circHMGCS1-MUT: 5’- GACGTCGTGTGCGTCGGTGCTAAGCTTCACAGATAC-3’.
Western blot analysis
Request a detailed protocolWestern blot was performed following previously described procedures (Xu et al., 2022). Protein extraction from cells or tissues was carried out using protein lysis buffer containing protease and phosphatase inhibitor cocktail (Beyotime, China). The concentrations of the protein lysates were determined using the Beyotime BCA Protein Assay Kit (Beyotime, China) according to the manufacturer’s instructions. Equal quantities of proteins (20 μg/lane) were loaded onto 8% or 10% SDS-PAGE gels and subsequently transferred to PVDF membranes (Millipore, USA). The membranes were then incubated with primary antibodies overnight at 4 °C. The primary antibodies used were as follows: anti-ARG1 (diluted 1:1000; Abcam, ab133543), anti-ARG2 (diluted 1:1000; Abcam, ab264066), anti-ET-1 (diluted 1:1000; Abcam, ab2786), anti-VCAM1 (diluted 1:1000; Abcam, ab134047), anti-ICAM1 (diluted 1:1000; Abcam, ab222736), anti-AGO2(diluted 1:2000; proteintech, 67934–1-Ig), anti-β-ACTIN (diluted 1:2000; Abcam, ab8226), and anti-GAPDH (diluted 1:2000; Abcam, ab8245). After incubation with the corresponding secondary antibodies for 2 hr at room temperature, the membranes were washed. Antibody binding was detected using an ECL detection reagent (Millipore, USA). Digital images were captured using a Gel DocTM XR +System with ImageLab software (Bio-Rad, USA). The quantification of the labeled bands was performed using ImageJ 1.55.
RNA fluorescent in situ hybridization (RNA-FISH)
Request a detailed protocolThe RNA-FISH assay was conducted in HUVECs. Cy3-labeled circHMGCS1 probe and FAM-labeled MIR4521 probe were custom-designed and synthesized by RiboBio (China). The signals emanating from the probes were detected using the Ribo FISH Kit (RiboBio) in accordance with the manufacturer’s protocol. Briefly, frozen sections were fixed with 4% paraformaldehyde for 10 min, followed by PBS washing. The fixed sections were treated with PBS containing 0.5% triton X-100 at 4 °C for 5 min, followed by incubation in pre-dehydration buffer at 37 °C for 30 min. Subsequently, probes targeting circHMGCS1 and MIR4521 were applied to the sections. Hybridization was performed in a humid chamber at 37 °C overnight. Post-hybridization washing was initially conducted with 4×saline sodium citrate containing 0.1% tween-20 at 42 °C, followed by further washing with 2×saline sodium citrate at 42 °C to eliminate non-specific and repetitive RNA hybridization. The slides were counterstained with DAPI (beyotime, China) and examined using an Olympus FV1200 (Olympus, Japan) confocal microscopy system. The mean fluorescent intensity (MFI) was determined using Image J software and subjected to statistical analysis. The probe sequences are detailed as follows: Cy3-circHMGCS1: 5’-UCCCACGAAGCCCUCAGUUGCUAUG-3’, FAM-MIR4521: 5’-UUUGACUCGUGUCCUGAAGGAAUCG-3’.
Luciferase reporter assay
Request a detailed protocolThe complete sequence of circHMGCS1 and a mutant circHMGCS1 sequence lacking the miRNA binding site were chemically synthesized. These sequences were inserted into the Nhel and SaII sites of the pmirGLO vector to generate expression vectors denoted as circHMGCS1-WT and circHMGCS1-MUT. Transfection was carried out using lipofectamine 2000 (Invitrogen, USA) with 50 nM MIR4521 mimics, MIR-NC, MIR4521 inhibitor, and MIR-NC inhibitor. HEK293T cells were seeded in 96-well plates and cultured until reaching 50%–70% confluence prior to transfection. After 48 hr of transfection, cells were harvested, and luciferase activity was quantified utilizing the Dual Luciferase Reporter Gene Assay Kit (Yeason, China). The pmirGLO vector expressing Renilla luciferase was utilized as an internal control for transfection, while the empty pmirGLO vector served as the negative control. Firefly luciferase activity of the pmirGLO vector was normalized with Renilla luciferase activity for comparison. Luciferase reporter assay was also used to investigate the regulation of MIR4521 on the expression of its target genes. For ARG1 and MIR4521, either wild-type or mutant ARG1 3’UTR fragments (433 bp) were inserted into NheI/SalI restriction sites of pmirGLO. One μg plasmids of ARG1 3’UTR-WT and ARG1 3’UTR-MUT, 50 nM MIR4521 mimics, MIR-NC, MIR4521 inhibitor and MIR-NC inhibitor were transfected. Luciferase activity was evaluated 48 hr post-transfection using the Dual Luciferase Reporter Gene Assay Kit (Yeason).
Histological and morphometric analysis
Request a detailed protocolAortic tissues were prepared by fixing them in 4% paraformaldehyde in PBS and subsequently embedding them in paraffin. The resulting paraffin-embedded tissues were then sectioned into 4-μm-thick slices. Hematoxylin-eosin (H&E) staining was performed on these sections to visualize the vascular tissue structures and assess the degree of thickness in the thoracic aorta. The average thickness of five randomly selected regions of the thoracic aorta was measured using OlyVIA software (Olympus, Japan).
Statistical analysis
Request a detailed protocolStatistical analyses were conducted using Prism software (GraphPad 8.0). Data are presented as mean ± standard deviation (SD) unless otherwise stated. Prior to statistical analysis, the normality of data distribution was evaluated using the Shapiro-Wilk test. For comparisons between the two groups, an unpaired two-tailed Student’s t-test was used. When comparing more than the two groups, either a one-way or two-way analysis of variance (ANOVA) was conducted, followed by the Bonferroni multiple comparison post hoc test. Group differences were considered statistically significant at a p-value of <0.05. The level of significance was indicated by asterisks, with *, **, and *** denoting p-values lower than 0.05, 0.01, and 0.001, respectively.
Data availability
Sequencing data have been deposited in GEO under accession codes GSE237295 and GSE237597, All data generated or analysed during this study are included in the manuscript and supporting files. Source data files for gels and blots are provided in the manuscript and supporting files.
-
NCBI Gene Expression OmnibusID GSE237295. The Circular RNA HMGCS1 Sponges miR-4521 to Aggravate Diabetes-Induced Vascular Endothelial Dysfunction Through Upregulating ARG1.
-
NCBI Gene Expression OmnibusID GSE237597. The Human Circular RNA CircHMGCS1 Sponges miR-4521 to Aggravates Diabetes-Induced Vascular Endothelial Dysfunction Through Upregulating ARG1.
References
-
Arginase: A multifaceted enzyme important in health and diseasePhysiological Reviews 98:641–665.https://doi.org/10.1152/physrev.00037.2016
-
Hyperglycemia inhibits endothelial nitric oxide synthase activity by posttranslational modification at the Akt siteThe Journal of Clinical Investigation 108:1341–1348.https://doi.org/10.1172/JCI11235
-
Endothelial cell metabolism in normal and diseased vasculatureCirculation Research 116:1231–1244.https://doi.org/10.1161/CIRCRESAHA.116.302855
-
Non-coding RNAs in human diseaseNature Reviews. Genetics 12:861–874.https://doi.org/10.1038/nrg3074
-
Regulation of microRNA function in animalsNature Reviews. Molecular Cell Biology 20:21–37.https://doi.org/10.1038/s41580-018-0045-7
-
The changing face of diabetes complicationsThe Lancet. Diabetes & Endocrinology 4:537–547.https://doi.org/10.1016/S2213-8587(16)30010-9
-
Circular RNA-ZNF532 regulates diabetes-induced retinal pericyte degeneration and vascular dysfunctionThe Journal of Clinical Investigation 130:3833–3847.https://doi.org/10.1172/JCI123353
-
Arginase inhibition mediates cardioprotection during ischaemia-reperfusionCardiovascular Research 85:147–154.https://doi.org/10.1093/cvr/cvp303
-
cutPrimers: A new tool for accurate cutting of primers from reads of targeted next generation sequencingJournal of Computational Biology 24:1138–1143.https://doi.org/10.1089/cmb.2017.0096
-
HISAT: a fast spliced aligner with low memory requirementsNature Methods 12:357–360.https://doi.org/10.1038/nmeth.3317
-
The biogenesis, biology and characterization of circular RNAsNature Reviews. Genetics 20:675–691.https://doi.org/10.1038/s41576-019-0158-7
-
Fast gapped-read alignment with Bowtie 2Nature Methods 9:357–359.https://doi.org/10.1038/nmeth.1923
-
Hallmarks of endothelial cell metabolism in health and diseaseCell Metabolism 30:414–433.https://doi.org/10.1016/j.cmet.2019.08.011
-
Short intronic repeat sequences facilitate circular RNA productionGenes & Development 28:2233–2247.https://doi.org/10.1101/gad.251926.114
-
Super enhancer-associated circular rna-circkrt4 regulates hypoxic pulmonary artery endothelial cell dysfunction in miceArteriosclerosis, Thrombosis, and Vascular Biology 43:1179–1198.https://doi.org/10.1161/ATVBAHA.122.318842
-
Non-coding RNAs as drug targetsNature Reviews. Drug Discovery 16:167–179.https://doi.org/10.1038/nrd.2016.117
-
Oxidative stress and cardiovascular risk: obesity, diabetes, smoking, and pollution: part 3 of a 3-part seriesJournal of the American College of Cardiology 70:230–251.https://doi.org/10.1016/j.jacc.2017.05.043
-
Human circular RNA‑0054633 regulates high glucose‑induced vascular endothelial cell dysfunction through the microRNA‑218/roundabout 1 and microRNA‑218/heme oxygenase‑1 axesInternational Journal of Molecular Medicine 42:597–606.https://doi.org/10.3892/ijmm.2018.3625
-
Arginase inhibitors: A rational approach over one centuryMedicinal Research Reviews 37:475–513.https://doi.org/10.1002/med.21419
-
CircSERPINE2 protects against osteoarthritis by targeting miR-1271 and ETS-related geneAnnals of the Rheumatic Diseases 78:826–836.https://doi.org/10.1136/annrheumdis-2018-214786
-
Gene regulation by long non-coding RNAs and its biological functionsNature Reviews. Molecular Cell Biology 22:96–118.https://doi.org/10.1038/s41580-020-00315-9
-
Breast cancer-derived GM-CSF regulates arginase 1 in myeloid cells to promote an immunosuppressive microenvironmentThe Journal of Clinical Investigation 131:e145296.https://doi.org/10.1172/JCI145296
-
Regulation of microRNA biogenesis and its crosstalk with other cellular pathwaysNature Reviews. Molecular Cell Biology 20:5–20.https://doi.org/10.1038/s41580-018-0059-1
-
Mechanistic pathogenesis of endothelial dysfunction in diabetic nephropathy and retinopathyFrontiers in Endocrinology 13:816400.https://doi.org/10.3389/fendo.2022.816400
-
CircRNA DICAR as a novel endogenous regulator for diabetic cardiomyopathy and diabetic pyroptosis of cardiomyocytesSignal Transduction and Targeted Therapy 8:99.https://doi.org/10.1038/s41392-022-01306-2
-
Global aetiology and epidemiology of type 2 diabetes mellitus and its complicationsNature Reviews. Endocrinology 14:88–98.https://doi.org/10.1038/nrendo.2017.151
-
Erythrocytes from patients with type 2 diabetes induce endothelial dysfunction via arginase IJournal of the American College of Cardiology 72:769–780.https://doi.org/10.1016/j.jacc.2018.05.052
Article and author information
Author details
Funding
The National Natural Science Foundation of China (32172192)
- Wei Chen
The National Natural Science Foundation of China (2021C02018)
- Wei Chen
The funders had no role in study design, data collection and interpretation, or the decision to submit the work for publication.
Acknowledgements
The authors thank core facilities of medicine in Zhejiang university for technical support. Funding: This work was supported by the National Natural Science Foundation of China (32172192) and Zhejiang Provincial Key R&D Program of China (2021C02018).
Ethics
All animal experiments were conducted in strict accordance with the guidelines and laws governing the use and care of laboratory animals in China (GB/T 35892-2018 and GB/T 35823-2018) and NIH Guide for the Care and Use of Laboratory Animals. The experimental procedures involving animals were performed at the Animal Experiment Center of Zhejiang Chinese Medical University in Hangzhou, China. The animal protocol was conducted in compliance with the guidelines set forth by the Ethics Committee for Laboratory Animal Care at Zhejiang Chinese Medical University (Approval No. 2022101345).
Version history
- Preprint posted:
- Sent for peer review:
- Reviewed Preprint version 1:
- Reviewed Preprint version 2:
- Version of Record published:
Cite all versions
You can cite all versions using the DOI https://doi.org/10.7554/eLife.97267. This DOI represents all versions, and will always resolve to the latest one.
Copyright
© 2024, Zhang et al.
This article is distributed under the terms of the Creative Commons Attribution License, which permits unrestricted use and redistribution provided that the original author and source are credited.
Metrics
-
- 414
- views
-
- 47
- downloads
-
- 0
- citations
Views, downloads and citations are aggregated across all versions of this paper published by eLife.
Download links
Downloads (link to download the article as PDF)
Open citations (links to open the citations from this article in various online reference manager services)
Cite this article (links to download the citations from this article in formats compatible with various reference manager tools)
Further reading
-
- Cell Biology
- Neuroscience
The claustrum complex is viewed as fundamental for higher-order cognition; however, the circuit organization and function of its neuroanatomical subregions are not well understood. We demonstrated that some of the key roles of the CLA complex can be attributed to the connectivity and function of a small group of neurons in its ventral subregion, the endopiriform (EN). We identified a subpopulation of EN neurons by their projection to the ventral CA1 (ENvCA1-proj. neurons), embedded in recurrent circuits with other EN neurons and the piriform cortex. Although the ENvCA1-proj. neuron activity was biased toward novelty across stimulus categories, their chemogenetic inhibition selectively disrupted the memory-guided but not innate responses of mice to novelty. Based on our functional connectivity analysis, we suggest that ENvCA1-proj. neurons serve as an essential node for recognition memory through recurrent circuits mediating sustained attention to novelty, and through feed-forward inhibition of distal vCA1 neurons shifting memory-guided behavior from familiarity to novelty.
-
- Cell Biology
- Computational and Systems Biology
Induced pluripotent stem cell (iPSC) technology is revolutionizing cell biology. However, the variability between individual iPSC lines and the lack of efficient technology to comprehensively characterize iPSC-derived cell types hinder its adoption in routine preclinical screening settings. To facilitate the validation of iPSC-derived cell culture composition, we have implemented an imaging assay based on cell painting and convolutional neural networks to recognize cell types in dense and mixed cultures with high fidelity. We have benchmarked our approach using pure and mixed cultures of neuroblastoma and astrocytoma cell lines and attained a classification accuracy above 96%. Through iterative data erosion, we found that inputs containing the nuclear region of interest and its close environment, allow achieving equally high classification accuracy as inputs containing the whole cell for semi-confluent cultures and preserved prediction accuracy even in very dense cultures. We then applied this regionally restricted cell profiling approach to evaluate the differentiation status of iPSC-derived neural cultures, by determining the ratio of postmitotic neurons and neural progenitors. We found that the cell-based prediction significantly outperformed an approach in which the population-level time in culture was used as a classification criterion (96% vs 86%, respectively). In mixed iPSC-derived neuronal cultures, microglia could be unequivocally discriminated from neurons, regardless of their reactivity state, and a tiered strategy allowed for further distinguishing activated from non-activated cell states, albeit with lower accuracy. Thus, morphological single-cell profiling provides a means to quantify cell composition in complex mixed neural cultures and holds promise for use in the quality control of iPSC-derived cell culture models.