Tardigrades: Surviving extreme radiation
When you think about the toughest animal in the world, you might think of a lion or tiger. But a less well-known contender for this title is a tiny animal known as a tardigrade, which is renowned for surviving extreme conditions. This includes being frozen, heated past the boiling point of water, completely dried out, exposed to the vacuum of outer space, and even being bombarded with extremely high levels of ionizing radiation (Kinchin, 1994; Jönsson, 2019).
How tardigrades endure these extremes is one of the prevailing mysteries of physiology. Now, in eLife, Jean-Paul Concordet and Anne de Cian from the Muséum National d'Histoire Naturelle and colleagues – including Marwan Anoud, Emmanuelle Delagoutte and Quentin Helleu as joint first authors – report new insights into how tardigrades survive exposure to ionizing radiation (Anoud et al., 2024).
Ionizing radiation typically damages cells by causing their DNA to fragment (Téoule, 1987). In the past, it was thought that organisms capable of surviving extreme doses of radiation, like tardigrades, might do so by blocking and preventing the radiation from harming their DNA. A previous report suggests that tardigrades utilize a protein known as Dsup to prevent DNA damage during radiation exposure (Hashimoto et al., 2016). However, Anoud et al. found that tardigrades accumulate the same amount of DNA damage as organisms and cells which are intolerant to radiation, such as human cells grown in a dish (Mohsin Ali et al., 2004). If tardigrades do not survive ionizing radiation by directly blocking DNA damage, how do they endure such an insult?
To investigate, the team (who are based at various institutes in France and Italy) examined three species of tardigrade, using a technique known as RNA sequencing, to see which genes are switched on when exposed to ionizing radiation. They also tested tardigrades exposed to bleomycin – a drug that mimics the effects of radiation by creating double-stranded breaks in DNA.
Anoud et al. found that tardigrades upregulated the expression of genes involved in the DNA repair machinery that is common across many life forms, ranging from single-celled organisms to humans. The DNA damage the tardigrades initially accumulated following radiation or treatment with bleomycin also gradually disappeared after the exposure. Overall, these results strongly suggest that to cope with the DNA damage caused by ionizing radiation, tardigrades mount a robust set of repair mechanisms to help stitch their shattered genome back together.
In addition to seeing that DNA repair machinery is upregulated following ionizing radiation, Anoud et al. identified a new gene only present in tardigrades, which encodes a protein they named TDR1 (short for tardigrade DNA repair protein 1). Further experiments revealed that TDR1 can enter the cell nucleus and bind to DNA. This may be due to conserved portions of TDR1, which are largely positively charged, electrostatically interacting with negatively charged DNA. Moreover, at high concentrations, TDR1 not only binds to DNA, but forms aggregates in a concentration-dependent manner. Finally, Anoud et al. found that introducing the gene for TDR1 into healthy human cells reduced the amount of DNA damage caused by bleomycin, indicating that the TDR1 protein helps with DNA repair (Figure 1).
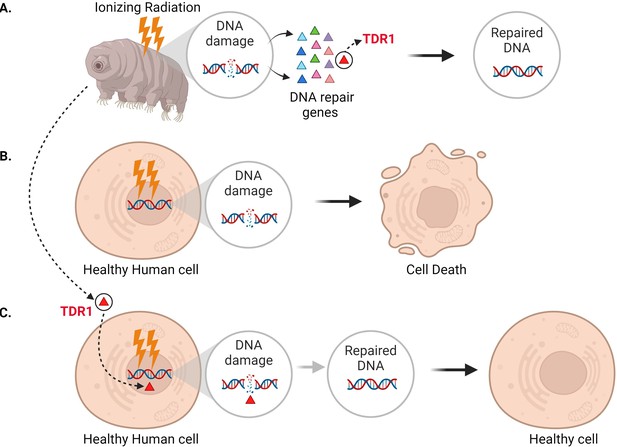
Expressing the tardigrade protein TDR1 in human cells increases DNA damage repair.
(A) In tardigrades, exposure to ionizing radiation or the drug bleomycin leads to DNA damage, such as double-stranded breaks (circle inset). This switches on various genes (triangles) that repair the genome, including the gene that codes for a protein called TDR1 (red triangle). (B) Bleomycin treatment (represented as lightning bolts) also causes double-stranded DNA breaks in human cells. However, these cannot be efficiently repaired, resulting in cell death. (C) Anoud et al. found that introducing the gene for TDR1 to the genome of human cells increases their ability to repair DNA damage and their chance of survival.
© 2024, BioRender Inc. Figure 1 was created using BioRender, and is published under a CC BY-NC-ND license. Further reproductions must adhere to the terms of this license.
While it is still unclear exactly how tardigrades fix DNA damage, this study suggests that their ability to survive extreme radiation is related to their strong DNA repair ability, which TDR1 likely plays a crucial role in. Anoud et al. found that TDR1 did not accumulate at DNA damage sites like some other repair proteins (Rothkamm et al., 2015). Instead, they propose that the protein mends DNA by binding to it and forming aggregates which compact the fragmented DNA and help maintain the organization of the damaged genome.
However, more research is needed to fully understand the mechanism responsible for TDR1 and other proteins helping tardigrades to survive ionizing radiation. Ultimately, knowing how these tiny organisms efficiently repair their DNA could lead to novel strategies for protecting human cells from radiation damage, which could benefit cancer treatment and space exploration.
References
-
DNA damage foci: Meaning and significanceEnvironmental and Molecular Mutagenesis 56:491–504.https://doi.org/10.1002/em.21944
-
Radiation-induced DNA damage and its repairInternational Journal of Radiation Biology and Related Studies in Physics, Chemistry, and Medicine 51:573–589.https://doi.org/10.1080/09553008414552111
Article and author information
Author details
Publication history
Copyright
© 2024, Swamy and Boothby
This article is distributed under the terms of the Creative Commons Attribution License, which permits unrestricted use and redistribution provided that the original author and source are credited.
Metrics
-
- 1,948
- views
-
- 118
- downloads
-
- 1
- citations
Views, downloads and citations are aggregated across all versions of this paper published by eLife.
Download links
Downloads (link to download the article as PDF)
Open citations (links to open the citations from this article in various online reference manager services)
Cite this article (links to download the citations from this article in formats compatible with various reference manager tools)
Further reading
-
- Cell Biology
- Chromosomes and Gene Expression
During oncogene-induced senescence there are striking changes in the organisation of heterochromatin in the nucleus. This is accompanied by activation of a pro-inflammatory gene expression programme – the senescence-associated secretory phenotype (SASP) – driven by transcription factors such as NF-κB. The relationship between heterochromatin re-organisation and the SASP has been unclear. Here, we show that TPR, a protein of the nuclear pore complex basket required for heterochromatin re-organisation during senescence, is also required for the very early activation of NF-κB signalling during the stress-response phase of oncogene-induced senescence. This is prior to activation of the SASP and occurs without affecting NF-κB nuclear import. We show that TPR is required for the activation of innate immune signalling at these early stages of senescence and we link this to the formation of heterochromatin-enriched cytoplasmic chromatin fragments thought to bleb off from the nuclear periphery. We show that HMGA1 is also required for cytoplasmic chromatin fragment formation. Together these data suggest that re-organisation of heterochromatin is involved in altered structural integrity of the nuclear periphery during senescence, and that this can lead to activation of cytoplasmic nucleic acid sensing, NF-κB signalling, and activation of the SASP.
-
- Chromosomes and Gene Expression
- Evolutionary Biology
Gene regulation is essential for life and controlled by regulatory DNA. Mutations can modify the activity of regulatory DNA, and also create new regulatory DNA, a process called regulatory emergence. Non-regulatory and regulatory DNA contain motifs to which transcription factors may bind. In prokaryotes, gene expression requires a stretch of DNA called a promoter, which contains two motifs called –10 and –35 boxes. However, these motifs may occur in both promoters and non-promoter DNA in multiple copies. They have been implicated in some studies to improve promoter activity, and in others to repress it. Here, we ask whether the presence of such motifs in different genetic sequences influences promoter evolution and emergence. To understand whether and how promoter motifs influence promoter emergence and evolution, we start from 50 ‘promoter islands’, DNA sequences enriched with –10 and –35 boxes. We mutagenize these starting ‘parent’ sequences, and measure gene expression driven by 240,000 of the resulting mutants. We find that the probability that mutations create an active promoter varies more than 200-fold, and is not correlated with the number of promoter motifs. For parent sequences without promoter activity, mutations created over 1500 new –10 and –35 boxes at unique positions in the library, but only ~0.3% of these resulted in de-novo promoter activity. Only ~13% of all –10 and –35 boxes contribute to de-novo promoter activity. For parent sequences with promoter activity, mutations created new –10 and –35 boxes in 11 specific positions that partially overlap with preexisting ones to modulate expression. We also find that –10 and –35 boxes do not repress promoter activity. Overall, our work demonstrates how promoter motifs influence promoter emergence and evolution. It has implications for predicting and understanding regulatory evolution, de novo genes, and phenotypic evolution.