Dopamine: Making memories
Dopamine is often referred to as the ‘feel-good’ neurotransmitter. Whether it is the satisfaction of a delicious meal, the thrill of success, or the drive to accomplish a goal – dopamine is central to our ability to experience pleasure, stay motivated and feel rewarded (Salamone et al., 2003; Schultz, 1997). However, it is also vital to how we learn and form lasting memories (Shohamy and Adcock, 2010; Takeuchi et al., 2016). So, how exactly does this neurotransmitter help lock memories in place?
When something new is learned, the synapses involved in storing the information become stronger through a process known as long-term potentiation (LTP), which relies on increased protein production. This results in the memory being more stable and easier to retrieve (Santini et al., 2014). Previous research, including work conducted by the research group led by Ole Paulsen, has shown that dopamine is critical for LTP, and that it can also regulate protein synthesis in neurons in the hippocampus, a brain region important for memory (Smith et al., 2005; Fuchsberger et al., 2022). Now, in eLife, Ole Paulsen and colleagues – including Tanja Fuchsberger as first author – report having identified a unique signaling pathway by which dopamine triggers the protein synthesis required to form long-term memories (Fuchsberger et al., 2024).
First, the team (who are based at the University of Cambridge and the MRC Laboratory of Molecular Biology) used a labelling technique to detect newly synthesized proteins in slices of the mouse hippocampus. This revealed that when dopamine was present, protein production significantly increased in CA1 neurons important for memory formation.
Further experiments showed that dopamine could transform long-term depression – a process that usually weakens synapses – into LTP, effectively converting weak synaptic connections into stronger ones. However, when protein synthesis was blocked, this ability disappeared. When dopamine was applied during neuronal stimulation, the synapses not only maintained their strength but grew even stronger, suggesting neuronal activation also contributes to the dopamine-induced increase in protein synthesis.
Fuchsberger et al. found that this strengthening of synaptic connections was mediated by two dopamine receptors (D1 and D5) that trigger a cascade of signals inside neurons. This includes activating enzymes known as adenylate cyclases, which simulate two other signaling molecules (cAMP and PKA), ultimately leading to a boost in protein production (Figure 1; Sassone-Corsi, 2012).
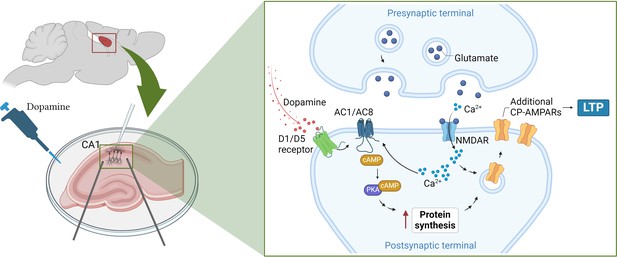
How dopamine increases synaptic strength in the hippocampus.
(Left) To test the effects of dopamine on long-term potentiation (LTP), Fuchsberger et al. injected the neurotransmitter into slices of the hippocampus (pink) and recorded the electrical activity of CA1 neurons (white electrode). (Right inset) They found that dopamine triggers the D1/D5 receptor (green) to activate a signaling pathway which includes the enzymes AC1/AC8 (dark blue), cAMP (brown) and PKA (purple). This leads to the production of more proteins that support the strengthening of the synapse and long-term potentiation. At the same time, neurotransmitters released from the pre-synaptic terminal, such as glutamate (dark blue circles), stimulate proteins on the post-synaptic terminal, such as NMDA receptors (light blue), to transmit calcium ions (Ca2+; light blue small circles) into the neuron. The calcium ions also activate AC1/AC8, ensuring that dopamine-induced protein synthesis is paired with neural activation. This increases the production of GluA1, a type of subunit that can assemble to form calcium-permeable AMPA receptors (yellow), which Fuchsberger et al. show to be essential for dopamine-induced LTP. AC1/AC8: adenylate cyclase 1/8; cAMP: cyclic adenosine monophosphate; PKA: protein kinase A. Figure 1 was created with BioRender.com.
Genetically removing two types of adenylate cyclase enzymes – called AC1 and AC8 – from CA1 neurons led to dopamine-induced LTP being completely absent. These enzymes are also activated by calcium ions that flood into the synapse during neuronal activation, suggesting that they couple neuronal activity and dopamine signaling in order to induce protein synthesis (Figure 1).
Fuchsberger et al. found that dopamine increased the production of the protein GluA1, a subunit found in AMPA receptors which support LTP. However, the neurotransmitter had no effect on GluA2, another type of subunit found in AMPA receptors.
AMPA receptors formed of only GluA1 subunits have captivated researchers due to their unique structures and signaling properties (Zhang et al., 2023), and the fact that, unlike AMPA receptors featuring other subunits, they allow calcium ions to pass through them (Park et al., 2019). Fuchsberger et al. discovered that when AMPA receptors containing just GluA1 were blocked, dopamine could no longer strengthen synaptic connections, suggesting that these calcium-permeable receptors are needed for dopamine-induced LTP (Figure 1).
These findings offer a fresh perspective on how dopamine influences, not only our motivation and mood, but also our ability to learn and remember. They show which signaling pathways and molecules allow dopamine to induce the protein synthesis required for long-term memory. This opens up exciting possibilities for targeting dopamine pathways in therapies designed to improve memory and learning, particularly for conditions like Alzheimer’s disease where memory formation is impaired. Modulating the effect of dopamine could also help reinforce memory in individuals experiencing cognitive decline.
One of the key challenges ahead is to fully understand the molecular mechanisms and cell-type specificity of dopamine’s influence on protein synthesis in neurons. Timing is also likely to play a crucial role, and understanding when dopamine is released in relation to neuronal activity may reveal critical periods for optimizing learning and memory processing. Future research exploring these temporal dynamics could shed light on the best approaches for harnessing dopamine’s full potential in enhancing memory and cognitive function.
References
-
On the role of calcium-permeable AMPARs in long-term potentiation and synaptic tagging in the rodent hippocampusFrontiers in Synaptic Neuroscience 11:4.https://doi.org/10.3389/fnsyn.2019.00004
-
Nucleus accumbens dopamine and the regulation of effort in food-seeking behavior: implications for studies of natural motivation, psychiatry, and drug abuseThe Journal of Pharmacology and Experimental Therapeutics 305:1–8.https://doi.org/10.1124/jpet.102.035063
-
Mechanisms of translation control underlying long-lasting synaptic plasticity and the consolidation of long-term memoryProgress in Molecular Biology and Translational Science 122:131–167.https://doi.org/10.1016/B978-0-12-420170-5.00005-2
-
The cyclic AMP pathwayCold Spring Harbor Perspectives in Biology 4:a011148.https://doi.org/10.1101/cshperspect.a011148
-
The phasic reward signal of primate dopamine neuronsAdvances in Pharmacology 42:686–690.https://doi.org/10.1016/s1054-3589(08)60841-8
-
Dopamine and adaptive memoryTrends in Cognitive Sciences 14:464–472.https://doi.org/10.1016/j.tics.2010.08.002
Article and author information
Author details
Publication history
Copyright
© 2024, Longo
This article is distributed under the terms of the Creative Commons Attribution License, which permits unrestricted use and redistribution provided that the original author and source are credited.
Metrics
-
- 662
- views
-
- 60
- downloads
-
- 0
- citations
Views, downloads and citations are aggregated across all versions of this paper published by eLife.
Download links
Downloads (link to download the article as PDF)
Open citations (links to open the citations from this article in various online reference manager services)
Cite this article (links to download the citations from this article in formats compatible with various reference manager tools)
Further reading
-
- Neuroscience
Motor systems operate over a range of frequencies and relative timing (phase). We studied the role of the hyperpolarization-activated inward current (Ih) in regulating these features in the pyloric rhythm of the stomatogastric ganglion (STG) of the crab, Cancer borealis, as temperature was altered from 11°C to 21°C. Under control conditions, rhythm frequency increased monotonically with temperature, while the phases of the pyloric dilator (PD), lateral pyloric (LP), and pyloric (PY) neurons remained constant. Blocking Ih with cesium (Cs+) phase advanced PD offset, LP onset, and LP offset at 11°C, and the latter two further advanced as temperature increased. In Cs+ the frequency increase with temperature diminished and the Q10 of the frequency dropped from ~1.75 to ~1.35. Unexpectedly in Cs+, the frequency dynamics became non-monotonic during temperature transitions; frequency initially dropped as temperature increased, then rose once temperature stabilized, creating a characteristic ‘jag’. Interestingly, these jags persisted during temperature transitions in Cs+ when the pacemaker was isolated by picrotoxin, although the temperature-induced change in frequency recovered to control levels. Overall, these data suggest that Ih plays an important role in maintaining smooth transitory responses and persistent frequency increases by different mechanisms in the pyloric circuitry during temperature fluctuations.
-
- Neuroscience
Channelrhodopsins (ChRs) are light-gated ion channels widely used to optically activate or silence selected electrogenic cells, such as individual brain neurons. Here, we describe identifying and characterizing a set of anion-conducting ChRs (ACRs) from diverse taxa and representing various branches of the ChR phylogenetic tree. The Mantoniella squamata ACR (MsACR1) showed high sensitivity to yellow-green light (λmax at 555 nm) and was further engineered for optogenetic applications. A single amino-acid substitution that mimicked red-light-sensitive rhodopsins like Chrimson shifted the photosensitivity 20 nm toward red light and accelerated photocurrent kinetics. Hence, it was named red and accelerated ACR, raACR. Both wild-type and mutant are capable optical silencers at low light intensities in mouse neurons in vitro and in vivo, while raACR offers a higher temporal resolution.