Spatiotemporal dynamics of PIEZO1 localization controls keratinocyte migration during wound healing
Abstract
Keratinocytes, the predominant cell type of the epidermis, migrate to reinstate the epithelial barrier during wound healing. Mechanical cues are known to regulate keratinocyte re-epithelialization and wound healing; however, the underlying molecular transducers and biophysical mechanisms remain elusive. Here, we show through molecular, cellular, and organismal studies that the mechanically activated ion channel PIEZO1 regulates keratinocyte migration and wound healing. Epidermal-specific Piezo1 knockout mice exhibited faster wound closure while gain-of-function mice displayed slower wound closure compared to littermate controls. By imaging the spatiotemporal localization dynamics of endogenous PIEZO1 channels, we find that channel enrichment at some regions of the wound edge induces a localized cellular retraction that slows keratinocyte collective migration. In migrating single keratinocytes, PIEZO1 is enriched at the rear of the cell, where maximal retraction occurs, and we find that chemical activation of PIEZO1 enhances retraction during single as well as collective migration. Our findings uncover novel molecular mechanisms underlying single and collective keratinocyte migration that may suggest a potential pharmacological target for wound treatment. More broadly, we show that nanoscale spatiotemporal dynamics of Piezo1 channels can control tissue-scale events, a finding with implications beyond wound healing to processes as diverse as development, homeostasis, disease, and repair.
Introduction
The skin, the largest organ of the body, serves as a barrier against a myriad of external insults while also performing important sensory and homeostatic functions. Cutaneous wounds interfere with all these functions and expose the body to an increased risk of infection, disease, and scar formation (Evans et al., 2013). During the repair of wounded skin, the migration of keratinocytes from the wound edge into the wound bed plays an essential step in re-establishing the epithelial barrier and restoring its protective functions (Kirfel and Herzog, 2004; Gantwerker and Hom, 2011). Accumulating evidence has shown that mechanical cues and cell-generated traction forces in keratinocytes play an important role in regulating the healing process and wound closure (Evans et al., 2013; Rosińczuk et al., 2016; Brugués, 2014; Hiroyasu et al., 2016; Huang et al., 2017; Ladoux and Mège, 2017). However, the molecular identity of keratinocyte mechanotransducers that control re-epithelialization remains unknown.
Cells are able to sense and detect mechanical forces, converting them into biochemical signals through the process of mechanotransduction. One class of mechanosensors utilized by cells are mechanically activated ion channels which offer the unique ability for cells to rapidly detect and transduce mechanical forces into electrochemical signals (Nourse and Pathak, 2017; Murthy et al., 2017). The Piezo1 ion channel has been shown to play an important role in a variety of cell types, and it regulates several key biological processes including vascular and lymphatic development, red blood cell volume regulation, stem cell fate, the baroreceptor response, cardiovascular homeostasis, cartilage mechanics, and others (Li et al., 2014; Ranade et al., 2014; Pathak et al., 2014; Rocio Servin-Vences et al., 2017; Zeng et al., 2018; Nonomura et al., 2018; Cahalan et al., 2015; Lee et al., 2014; Rode et al., 2017). Previous studies in MDCK cells and in zebrafish larvae have demonstrated the importance of the channel in homeostatic regulation of epithelial cell numbers (Gudipaty et al., 2017; Eisenhoffer et al., 2012). As yet, the role of Piezo1 in skin wound healing, an important epithelial function, has not been investigated. We asked whether PIEZO1 may function as a mechanosensor regulating keratinocyte re-epithelialization during the wound healing process. Here, we show that PIEZO1 activity increases cellular retraction, reducing the efficiency of keratinocyte migration and wound healing, and that inhibition of PIEZO1 results in faster wound healing in vitro and in vivo. The channel exhibits dynamic changes in its subcellular localization, concentrating at areas of the wound edge and causing local retraction at these regions.
Results
Reduced PIEZO1 accelerates wound healing
Analysis of Piezo channel mRNA expression in mouse tissues has previously shown that Piezo1 is highly expressed in skin, while Piezo2 is less abundant (Coste et al., 2010). To characterize PIEZO1 expression profile in skin, we used a reporter mouse expressing a promoter-less β-geo (β-gal and neomycin phosphotransferase) in-frame with a portion of the PIEZO1 channel (Ranade et al., 2014). LacZ staining of skin tissue from these reporter mice revealed a high expression of PIEZO1 in the epidermal layer of keratinocytes as well as in hair follicles (Figure 1A).
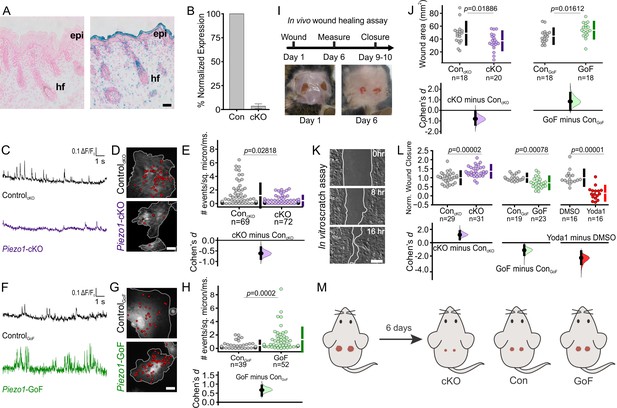
PIEZO1 is expressed in keratinocytes, produces Ca2+ flickers, and regulates skin wound healing.
(A) Representative images of LacZ stained Piezo1+/+ (left) and Piezo+/βGeo (right) skin sections from P2 (postnatal day 2) mice. Scale bar = 20 µm. epi, epidermis. hf, hair follicle. (B) qRT-PCR from primary neonatal keratinocytes of Piezo1 mRNA expression in Krt14+/+ Piezo1fl/fl (Con) and Krt14Cre/+;Piezo1fl/fl (conditional knockout [cKO]) mice. Data presented as the mean ± SEM. See also Figure 1—figure supplements 1–2. Data collected from two litters. (C) Representative examples of Ca2+ flickers recorded from ControlcKO (top) and Piezo1-cKO (bottom) keratinocytes. Traces show fluorescence ratio changes (ΔF/F0) from a Ca2+ flicker site, plotted against time. (D) Representative images of sites of Ca2+flickers (red dots) overlaid on images of keratinocytes isolated from ControlcKO (top) and littermate Piezo1-cKO (bottom) mice that are loaded with fluorescent Ca2+ indicator Cal-520AM. Gray line denotes cell boundary, Scale bar = 20 µm. (E) Cumming plot showing frequency of Ca2+ flickers in Piezo1-cKO and respective ControlcKO cells (p value calculated via Mann-Whitney test; Cohen’s d = −0.6175). n in E denotes the number of videos (i.e., unique fields of view imaged, each of which is composed of one or more cells). Videos were collected from four independent experiments from three litters. See also Figure 1—video 1. (F, G, H) Similar to C, D, E but using keratinocytes from ControlGoF (top) and Piezo1-GoF (bottom) mice (p value calculated via Mann-Whitney test; Cohen’s d = 0.6747). Videos were collected from four independent experiments from three litters. See also Figure 1—video 2. (I) Diagram of in vivo wound healing model (top) and representative wound images at days 1 and 6 (bottom). (J) Cumming plot showing wound area of Piezo1-cKO (left) and Piezo1-GoF (right) groups at day 6 relative to control (Con) littermates (p value calculated via two-sample t-test; Cohen’s d = −0.799 and 0.844, respectively). Control (Con) refers to the Cre-negative littermates in each group. n in J denotes number of wounds measured, with two wounds per animal. (K) Representative images of an in vitro scratch assay. White line represents the monolayer edge. Scale bar = 200 µm. (L) Cumming plot showing quantification of scratch wound closure in monolayers of keratinocytes isolated from: ControlcKO vs. Piezo1-cKO mice (left; p value calculated via two-sample t-test; Cohen’s d = 1.188; images from three independent experiments), ControlGoF vs. Piezo1-GoF mice (middle; p value calculated via two-sample t-test; Cohen’s d = −1.128; images from four independent experiments) or DMSO-treated vs 4 µM Yoda1-treated ControlcKO monolayers (right; p value calculated via Mann-Whitney test; Cohen’s d = −2.278; images from three independent experiments). n in L denotes the number of unique fields of view imaged. Data are normalized to the mean scratch closure of the corresponding control condition where one is the average closure distance of the control and 0 is no closure. See also Figure 1—figure supplement 3. (M) Schematic illustrating results from in vivo wound healing assay shown in I and J. Mice were wounded (left) and after 6 days, wounds of Piezo1-cKO mice healed more than Control, whereas wounds from Piezo1-GoF mice healed less. Vertical bars in upper Cumming plots denote mean ± s.d.
-
Figure 1—source data 1
PIEZO1 is expressed in keratinocytes, produces Ca2+ flickers, and regulates skin wound healing.
(Sheet 1) qRT-PCR data from primary neonatal keratinocytes of Piezo1 mRNA expression in Krt14+/+ Piezo1fl/fl (Con) and Krt14Cre/+;Piezo1fl/fl (conditional knockout [cKO]) mice seen in Figure 1B. (Sheet 2) Flicker fluorescence ratio changes (ΔF/F0) from a region of interest, plotted against time shown in Figure 1C from ControlcKO (left) and Piezo1-cKO (right) keratinocytes. (Sheet 3) Data plotted in Figure 1E showing frequency of Ca2+ flickers in Piezo1-cKO and respective ControlcKO cells. (Sheets 4, 5) Similar to Sheets 2, 3 but using keratinocytes from ControlGoF and Piezo1-GoF mice for data shown in Figure 1F,H. (Sheet 6) Data used for creating Cumming plot in Figure 1J showing wound area of Piezo1-cKO and Piezo1-GoF groups at day 6 relative to control (Con) littermates. (Sheet 7) Normalized plot data seen in Figure 1L showing scratch wound closure in monolayers of keratinocytes isolated from: ControlcKO vs. Piezo1-cKO mice, ControlGoF vs. Piezo1-GoF mice and DMSO-treated vs. 4 µM Yoda1-treated ControlcKO monolayers.
- https://cdn.elifesciences.org/articles/65415/elife-65415-fig1-data1-v2.xlsx
Since the global knockout of Piezo1 is embryonically lethal (Li et al., 2014; Ranade et al., 2014), we generated an epidermal-specific knockout mouse to investigate whether PIEZO1 plays a role in cutaneous wound healing. The Krt14Cre mouse line was crossed with Piezo1fl/fl mice (Cahalan et al., 2015) to generate Krt14Cre;Piezo1fl/fl mice (hereafter referred to as conditional knockout, cKO) which are viable, develop normally, and feature normal skin sections (Figure 1—figure supplement 1), consistent with observations by Moehring et al., 2020. qRT-PCR analysis using keratinocytes harvested from Piezo1-cKO and littermate control animals confirmed expression of Piezo1 and not Piezo2 in control mice (Figure 1—figure supplement 2), and showed that Piezo1 mRNA expression is efficiently abrogated in cells from cKO animals (Figure 1B). Furthermore, we also generated a Piezo1 gain-of-function (GoF) mouse line (Piezo1-GoF) which expresses the gain of function (GoF) Piezo1 mutation, R2482H (Ma et al., 2018), in keratinocytes.
To confirm functional change to PIEZO1 in mutant keratinocytes, we performed Ca2+ imaging using total internal reflection fluorescence (TIRF) microscopy of keratinocytes isolated from Piezo1 cKO, GoF, and their respective control (Cre-) littermates. We previously reported that in adherent cells Piezo1 produces Ca2+ flickers in response to cell-generated forces in the absence of external mechanical stimulation (Pathak et al., 2014; Ellefsen et al., 2019). Compared to littermate control (ControlcKO) cells, keratinocytes from Piezo1-cKO mice showed a 63% reduction in Ca2+ flickers, indicating that a majority of Ca2+ flickers arise from cell-generated activation of the PIEZO1 channel (Figure 1C, D, E, Figure 1—video 1). Piezo1-GoF cells displayed a nearly threefold increase in the frequency of Ca2+ flickers relative to littermate controls (ControlGoF) (Figure 1F, G, H. Figure 1—video 2), further supporting PIEZO1 as a key source of Ca2+ flickers. A difference in the frequency of Ca2+ flickers between the ControlcKO and ControlGoF cells was observed, likely arising from different genetic backgrounds of the two strains. For this reason, in all subsequent experiments, mutant keratinocytes are compared to littermate control cells of the same genetic background.
To investigate the function of PIEZO1 in keratinocytes in vivo, we generated full-thickness wounds on the dorsal skin of Piezo1 cKO, GoF, and their respective control littermates and assessed wound closure (Figure 1I). Six days post wounding, Piezo1-cKO mice displayed significantly smaller wound areas relative to their control littermates, while Piezo1-GoF mice showed larger wound areas, suggesting that increased channel activity leads to impaired rates of wound closure (Figure 1J).
To determine whether the effect on wound healing was caused by changes to the rate of keratinocyte re-epithelialization, we mimicked the in vivo wound healing paradigm in vitro. We generated scratch wounds in keratinocyte monolayers to trigger the re-epithelialization process and allowed the monolayers to migrate toward each other (Figure 1K). Scratches in monolayers of Piezo1-cKO keratinocytes closed faster than those from littermate ControlcKO cells (Figure 1L, left). Conversely, scratch closure in monolayers of Piezo1-GoF keratinocytes was significantly slower (Figure 1L, middle). Correspondingly, when the PIEZO1 agonist Yoda1 was added to healing ControlcKO monolayers at concentrations greater than 2 μM, scratch wound closure was also significantly impaired (Figure 1L, right, Figure 1—figure supplement 3A), further supporting PIEZO1 involvement in re-epithelialization. No effect on wound closure was observed when Piezo1-cKO monolayers were treated with Yoda1 indicating that inhibition of scratch closure is the result of PIEZO1 activity (Figure 1—figure supplement 3B). Collectively, our in vitro and in vivo data demonstrate that the PIEZO1 ion channel plays an important role in wound healing, with Piezo1 knockout accelerating the healing process (Figure 1M).
PIEZO1 regulates keratinocyte migration
To determine whether the differences in wound closure rates arise due to PIEZO1’s effect on keratinocyte motility during the re-epithelialization process, we captured migration dynamics of dissociated single keratinocytes from Piezo1-cKO mice. Isolated cells were sparsely seeded onto fibronectin-coated glass-bottom dishes and imaged over several hours using differential interference contrast (DIC) time-lapse imaging (Figure 2A, Figure 2—video 1). We tracked the position of individual cells in the acquired movies and analyzed the extracted cell migration trajectories using an open-source algorithm, DiPer (Gorelik and Gautreau, 2014). The time-lapse images and corresponding cell migration trajectories (Figure 2B; Figure 2—figure supplement 1) revealed that the migration patterns of Piezo1-cKO keratinocytes are distinct from their littermate control cells. To quantify cellular migration, we generated mean squared displacement (MSD) plots which provide a measure of the surface area explored by the cells, and is an indication of the overall efficiency of migration. Interestingly, Piezo1-cKO keratinocytes explored a larger area compared to littermate ControlcKO cells (Figure 2C).
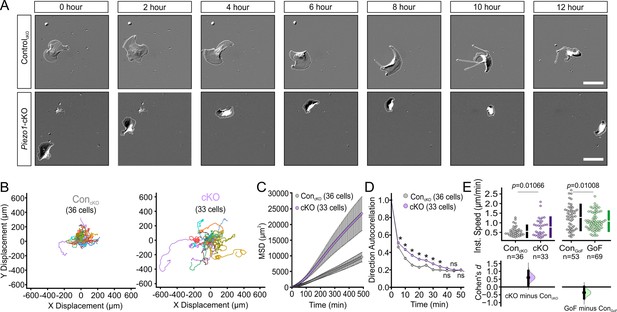
PIEZO1 mediates speed and direction during single cell keratinocyte migration.
(A) Representative differential interference contrast (DIC) images from time-lapse series of individual migrating keratinocytes isolated from ControlcKO (top) and respective Piezo1-cKO mice (bottom). Thin white lines denote the cell boundary. Scale bar = 25 µm. (B) Cell trajectories derived from tracking single keratinocytes during time-lapse experiments. Trajectories are shown with cell position at time point 0 normalized to the origin. See also Figure 2—figure supplement 1. (C) Mean squared displacement (MSD) analysis of ControlcKO and Piezo1-cKO keratinocytes tracked in B. Average MSD is plotted as a function of time. Error bars (SEM) are smaller than symbols at some points. (D) Average direction autocorrelation measurement of Piezo1-cKO and ControlcKO keratinocytes plotted as a function of time interval. * denotes a statistically significant difference, and ns denotes ‘not statistically significant’. From left to right: p = 2.0307 × 10–4, 5.75675 × 10–14, 3.18447 × 10–15, 5.34662 × 10–10, 1.72352 × 10–4, 1.34648 × 10–5, 0.01951, 0.13381, 0.61758 as determined by Kruskal-Wallis test. Plotted error bars (SEM) are smaller than symbols. (E) Quantitation of the average instantaneous speed from individual Piezo1-cKO keratinocytes (left) and Piezo1-GoF keratinocytes (right) relative to the respective control cells are shown in a Cumming plot (Cohen’s d = 0.6 [Piezo1-cKO]; d = −0.362 [Piezo1-GoF]; p values calculated via Kolmogorov-Smirnov test). n in B–E denotes the number of individually migrating cells tracked. See also Figure 2—figure supplements 2–3 and Figure 2 videos 1 and 2. Data are from three independent experiments from two litters for conditional knockout (cKO) and six independent experiments from five litters for gain-of-function (GoF). Bars in upper Cumming plots denote mean ± s.d.
-
Figure 2—source data 1
PIEZO1 mediates speed and direction during single cell keratinocyte migration.
(Sheets 1, 2) X,Y coordinates used to plot cell trajectories derived from tracking single (Sheet 1) control and (Sheet 2) conditional knockout (cKO) keratinocytes plotted in Figure 2B. (Sheet 3) Average mean squared displacement (MSD) analysis of ControlcKO and Piezo1-cKO keratinocytes plotted in Figure 2C. (Sheet 4) MSD for individual cells used to create averages found in Sheet 3. (Sheet 5) Average direction autocorrelation measurement of Piezo1-cKO and ControlcKO keratinocytes plotted as a function of time interval in Figure 2D. (Sheet 6) Quantitation of the average instantaneous speed from individual Piezo1-cKO keratinocytes and Piezo1-GoF keratinocytes relative to the respective control cells plotted in Figure 2E.
- https://cdn.elifesciences.org/articles/65415/elife-65415-fig2-data1-v2.xlsx
The MSD of a migrating cell is determined by two parameters: directional persistence (propensity of the cell to move in a straight line) and displacement rate (speed). To assess directional persistence, we performed direction autocorrelation analysis, a robust measure of migration directionality that, unlike the more commonly used directionality ratio analysis, is not confounded by differences in migration speed (Gorelik and Gautreau, 2014). The direction autocorrelation function for trajectories from Piezo1-cKO keratinocytes decayed slower than for littermate ControlcKO cells, indicative of fewer turns and a straighter trajectory (Figure 2D). The average instantaneous speed calculated by DiPer analysis was higher for Piezo1-cKO cells relative to littermate ControlcKO cells (Figure 2E). Thus, Piezo1-cKO keratinocytes migrate significantly faster and straighter. Similarly, we extracted cell migration trajectories from single migrating keratinocytes harvested from Piezo1-GoF and littermate ControlGoF mice (Figure 2—figure supplement 2, Figure 2—video 2). We observed no difference in the MSD plots of Piezo1-GoF keratinocytes and littermate ControlGoF cells (Figure 2—figure supplement 3). However, separating the data into directionality and speed indicated that Piezo1-GoF cells moved straighter (Figure 2—figure supplement 3) and slower (Figure 2E). Overall, our data demonstrate that PIEZO1 regulates keratinocyte migration, with channel activity resulting in slower migration speed. The effects on directionality were more complex, with both PIEZO1 knockout and a GoF mutation resulting in straighter trajectories.
PIEZO1 regulates cell shape and induces a polarized shape
To gain insights into how PIEZO1 may regulate cell migration, we visualized localization of endogenous PIEZO1 in single keratinocytes harvested from a Piezo1-tdTomato fusion knock-in reporter mouse (Ranade et al., 2014). Using this model, we previously reported punctate membrane localization of endogenous PIEZO1-tdTomato channels in neural stem/progenitor cells and mouse embryonic fibroblasts (Ellefsen et al., 2019). Here, we directly imaged endogenous PIEZO1-tdTomato’s subcellular localization in individual live, migrating keratinocytes using TIRF imaging and noticed higher PIEZO1 levels at the rear end of the cell (Figure 3A, Figure 3—video 1). This observation of PIEZO1-tdTomato enrichment at the rear of single migrating cells suggests that PIEZO1 may underlie cell polarization during migration.
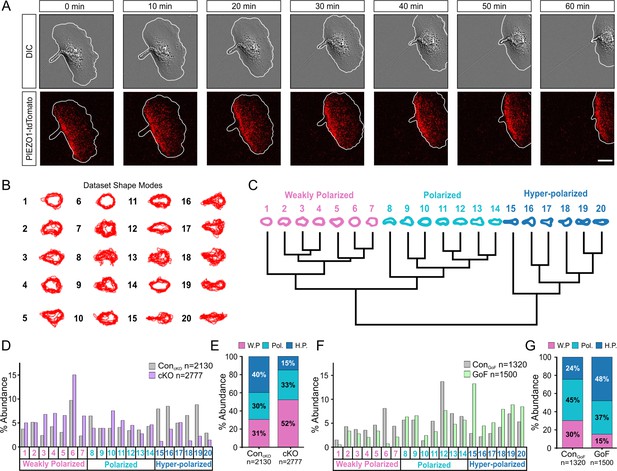
PIEZO1 activity promotes cell polarization.
(A) Time-lapse series of representative differential interference contrast (DIC) (top) and total internal reflection fluorescence (TIRF) (bottom) images illustrating the location of PIEZO1-tdTomato protein during keratinocyte migration. White lines denote the cell boundary. Scale bar = 15 µm. Images representative of four independent experiments from two litters. See also Figure 3—video 1. (B) Representative overlays of cell outlines segmented from ControlcKO, Piezo1-cKO, ControlGoF, and Piezo1-GoF single cell migration time-lapse images and classified into 20 representative shape modes using VAMPIRE (visually aided morpho-phenotyping image recognition), a machine learning algorithm designed for quantification of cellular morphological phenotypes. (C) Dendrogram showing the level of correlation between shape modes identified by VAMPIRE. Cell shape modes are classified into the morphologically distinct categories of weakly polarized (pink), polarized (blue), or hyper-polarized (dark blue). (D) Bar plots showing the distribution of cell shape modes of ControlcKO (gray; n denotes the number of images analyzed from seven cells and two independent experiments) and Piezo1-cKO (purple; n denotes the number of segmented shapes from 12 cells and two independent experiments) cells. (E) Stacked bar graphs indicating the proportion of weakly polarized (W.P.) (pink), polarized (Pol.) (blue), or hyper-polarized (H.P.) (dark blue) shape modes in ControlcKO (left) and Piezo1-cKO (right) cells. (F, G) Similar to D and E respectively but showing the distribution of cell shape modes for Piezo1-GoF (green; n denotes the number of shapes from nine cells and three independent experiments) and respective ControlGoF (gray; n denotes the number of shapes from eight cells and three independent experiments) cells during single cell migration time-lapse experiments. See also Figure 2 videos 1 and 2. Bars in D-G denote frequency.
-
Figure 3—source data 1
PIEZO1 activity promotes cell polarization.
(Sheet 1) Relative frequency data used to create bar plots showing the distribution of cell shape modes of ControlcKO and Piezo1-cKO cells in Figure 3D. (Sheet 2) Cumulative frequency used to create stacked bar graphs seen in Figure 3E indicating the proportion of weakly polarized, polarized, or hyper-polarized shape modes in ControlcKO and Piezo1-cKO cells. (Sheet 3) Shape mode output from VAMPIRE (visually aided morpho-phenotyping image recognition) used to create shape mode averages in Sheets 1 and 2. (Sheets 4, 5, 6) Similar to Sheets 1, 2, and 3 respectively but using Piezo1-GoF and ControlGoF cells for graphs in Figure 3F,G.
- https://cdn.elifesciences.org/articles/65415/elife-65415-fig3-data1-v2.xlsx
To determine whether PIEZO1 may be responsible for generating the polarized shape, we performed cellular morphometrics on the images obtained from the above time-lapse imaging of single cell migration. We used visually aided morpho-phenotyping image recognition (VAMPIRE) (Phillip et al., 2021), a high-throughput machine-learning algorithm that analyzes the morphology of individual cells in a population by quantifying shape modes of segmented cells and showing the level of correlation between the shape modes through a dendrogram (Figure 3B and C). VAMPIRE classification of the Piezo1-cKO and littermate ControlcKO keratinocytes into 20 shape modes revealed that Piezo1-cKO reduced the proportion of highly polarized shapes and increased the proportion of weakly polarized shapes relative to littermate ControlcKO keratinocytes (Figure 3D and E). On the other hand, the GoF mutation increased the frequency of polarized and hyper-polarized shapes at the expense of unpolarized or weakly polarized cell shapes (Figure 3F and G). Taken together, these results indicate that PIEZO1 activity promotes cell polarization. Based on imaging the localization of endogenous PIEZO1 channels in migrating cells, it appears that this may be mediated by regulation of the channel’s subcellular localization.
Dynamic PIEZO1 localization informs retraction events to regulate wound healing
We then examined a role for PIEZO1 localization in wounded cell monolayers. We generated a scratch wound in a confluent monolayer of Piezo1-tdTomato keratinocytes and imaged spatiotemporal dynamics of PIEZO1-tdTomato localization at the cell-substrate interface using TIRFM imaging together with DIC imaging over a period of several hours. We found that at some regions along the wound margin, PIEZO1-tdTomato was enriched in band-like structures (Figure 4A). Interestingly, this enrichment, which was observed a few hours after scratch generation (compare Figure 4—figure supplement 1 and Figure 4A), was also highly dynamic, such that it ebbed and flowed over the course of imaging (Figure 4—video 1).
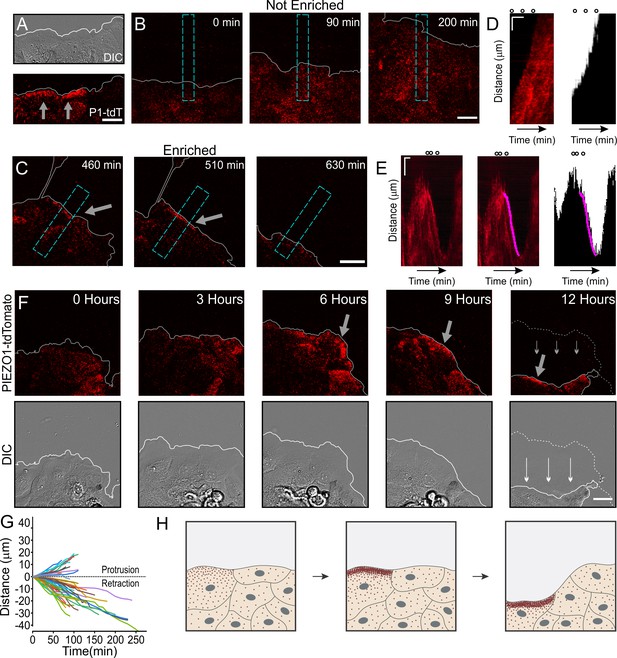
Dynamic PIEZO1 channel localization controls wound edge retraction.
(A) Representative differential interference contrast (DIC) (top) and total internal reflection fluorescence (TIRF) (bottom) image visualizing the location of PIEZO1-tdTomato (P1-tdT) protein in live, collectively migrating keratinocytes in an in vitro scratch assay. Gray lines denote the boundary of the cell monolayer. Gray arrows indicate regions of PIEZO1 enrichment. Scale bar = 20 μm. See also Figure 4—figure supplement 1. (B, C) Representative TIRF images taken from time-lapse image series of healing monolayers from PIEZO1-tdTomato keratinocytes highlighting fields of view in which PIEZO1-tdTomato is not enriched (B) and enriched (C) at the monolayer’s leading edge. Gray lines denote the boundary of the cell monolayer. Arrow indicates regions of enrichment. Scale bar = 20 μm. Blue dashed rectangles in D and E depict the regions used to generate kymographs in D and E. TIRF images were acquired every 10 min over a period of 16.6 hr. (D, E) Left panels: Representative kymographs illustrating PIEZO1-tdTomato puncta dynamics during the time-lapse series shown in B and C, respectively. Middle panel (for E only): Magenta line denotes periods of PIEZO1-tdTomato puncta enrichment at the wound edge, identified and tracked using the deep learning-based kymograph analysis software, Kymobutler. No Kymobutler tracks were detected in non-enriched regions (D). Right panels: Representative kymographs from binarized versions of DIC images corresponding to D and E, respectively, with the Kymobutler track output from the middle panel overlaid. The cell monolayer is represented in black, and white denotes cell-free space of the wounded area. Note the PIEZO1-tdTomato enrichment track correlates with periods of cell retraction. Scale bar = 10 μm. Time bar = 100 min. Black open circles on top represent the time-points of images shown in D and E. See also Figure 4—video 1 and Figure 4—video 2. (F) DIC (bottom) and TIRF (top) images during a time-lapse imaging series following scratch generation at 0 hr from a field of view showing sustained PIEZO1-tdTomato localization and marked monolayer retraction. Gray (top) and white (bottom) lines denote the boundary of the monolayer. Dotted line in the 12 hr image denotes the position of the monolayer at 6 hr; thin arrows indicate direction of monolayer movement during this period. Large gray arrow indicates region of PIEZO1 enrichment. Scale bar = 20 μm. See also Figure 4—video 3. (G) Plot showing 54 individual PIEZO1-tdTomato Kymobutler tracks from 25 kymographs collected from three independent experiments after normalizing the starting spatial and time coordinates of each track to the origin. (H) Schematic of a healing monolayer indicating distributed Piezo1 localization (red dots) following scratch generation (left), the development of areas of PIEZO1 enrichment (middle), and subsequent retraction of those areas (right).
-
Figure 4—source data 1
Dynamic PIEZO1 channel localization controls wound edge retraction.
(Sheet 1) PIEZO1-tdTomato Kymobutler tracks plotted in Figure 4G.
- https://cdn.elifesciences.org/articles/65415/elife-65415-fig4-data1-v2.xlsx
We asked whether regions displaying PIEZO1-tdTomato enrichment migrate differently from regions without enrichment. To systematically assess the relationship between PIEZO1-tdTomato enrichment and wound edge dynamics, we used kymographs to graphically represent PIEZO1-tdTomato position over the imaging period from regions that displayed PIEZO1-tdTomato enrichment at the wound edge (Figure 4C, E, Figure 4—video 1) and compared them to control fields of view that showed no such channel enrichment throughout the videos (Figure 4B, D, Figure 4—video 2). PIEZO1-tdTomato enrichment events at the wound edge appeared as linear streaks in the kymographs (Figure 4E, left panel) which could be objectively identified by Kymobutler, a deep-learning-based kymograph analysis software (Jakobs et al., 2019; Figure 4E, middle and right panels). For the kymographs from control fields of view (Figure 4B, Figure 4—video 2), Kymobutler did not detect any tracks and we did not observe retraction of the wound edge (Figure 4D). In fields of view that exhibited PIEZO1-tdTomato puncta enrichment at the wound edge, this channel enrichment was followed by a localized retraction of the wound edge (Figure 4C, Figure 4E, Figure 4—video 1). Kymobutler analysis of PIEZO1-tdTomato tracks overlaid on the DIC kymographs allowed examination of the migration dynamics of the wound edge in relation to Piezo1 enrichment (Figure 4E); 72% of these PIEZO1-tdTomato enrichment tracks displayed a negative slope corresponding to cell edge retraction and aligned with retraction events (Figure 4G). In some fields of view, PIEZO1 enrichment and the accompanying retraction lasted for shorter periods of time, and the periods without channel enrichment were accompanied by wound edge protrusion. In other cases, channel enrichment was maintained for several hours and was accompanied by a sustained and overt retraction of the wound edge throughout that period (Figure 4F, Figure 4—video 3). Thus, enrichment of PIEZO1-tdTomato puncta resulted in wound edge retraction (Figure 4H). Importantly, the rear end of single migrating cells, where PIEZO1 is found to localize (Figure 3A), is also the site of cellular retraction (Petrie et al., 2009; Yam, 2007), suggesting a general relationship between PIEZO1 localization and retraction.
PIEZO1 activation causes cellular retraction
To examine the relationship between PIEZO1 activity and cellular retraction, we examined the effect of chemical activation of PIEZO1 by Yoda1. We first focused on cellular dynamics of single, migrating ControlcKO keratinocytes by imaging at a high spatiotemporal resolution. Using DIC imaging, we monitored migrating keratinocytes at 5 second intervals under control conditions and after Yoda1 treatment (Figure 5A, Figure 5—video 1). Kymographs were used to visualize changes in the cell edge position over time. We observed that under control conditions, the cell edge displayed cycles of protrusion and retraction which was expected since cell migration is known to progress by iterative cycles of protrusion and retraction (Giannone et al., 2004; Giannone et al., 2007). PIEZO1 activation by 4 μM Yoda1 greatly affected these cycles and resulted in an extremely dynamic cell edge (Figure 5A, Figure 5—video 1). The frequency as well as the speed of cell edge retractions and protrusions increased upon Yoda1 treatment but resulted in a net cellular retraction over time (Figure 5A, Figure 5—figure supplement 1C), with some cells demonstrating drastic retraction with Yoda1 treatment (Figure 5—video 2). Piezo1-cKO keratinocytes did not show an increase in retraction upon treatment with 4 μM Yoda1 (Figure 5B and , Figure 5—video 3, Figure 5—figure supplement 1), demonstrating that Yoda1-induced increase in retraction is mediated by PIEZO1. Additionally, kymographs of Piezo1-GoF keratinocytes also showed an increase in cell edge dynamics compared to littermate ControlGoF cells further supporting PIEZO1’s role in retraction (Figure 5—figure supplement 1 and Figure 5—video 4).
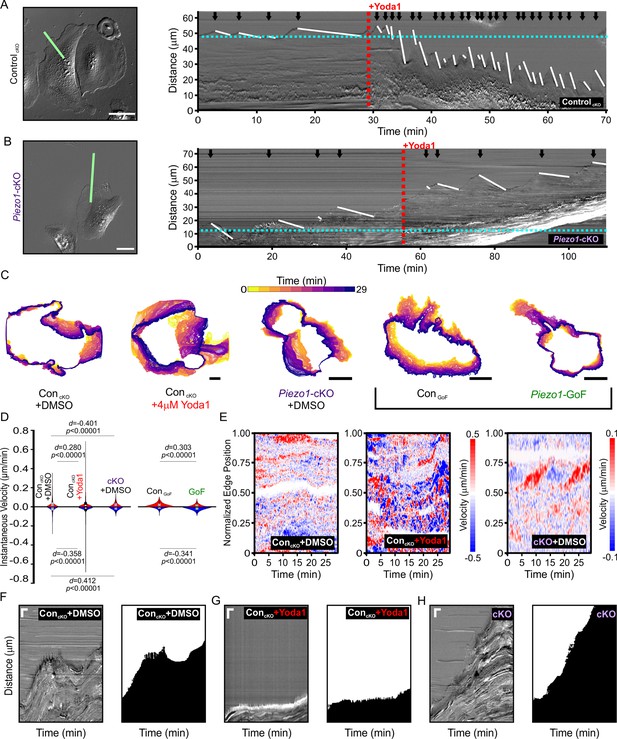
PIEZO1 activity promotes cellular retraction and increases edge velocity.
(A) Image still from a differential interference contrast (DIC) time-lapse series of ControlcKO keratinocytes (left). Scale bar = 50 µm. The green line denotes the representative region of interest used for generating kymograph (right). The red dotted line denotes the addition of 4 µM Yoda1. The black arrows indicate retraction events, and the slope of the descending white lines denotes the speed of retraction. Dotted blue line denotes starting position of the cell edge. See also Figure 5—figure supplement 1, Figure 5—video 1 and Figure 5—video 2. Images representative of six independent experiments from cells isolated from three litters. (B) Similar to A but using Piezo1-cKO keratinocytes with the same annotations. Scale bar = 25 µm. See also Figure 5—figure supplement 1, Figure 5—video 3 and Figure 5—video 4. Images representative of seven independent experiments from cells isolated from four litters. (C) Representative overlays of cell boundary outlines detected and segmented from DIC time-lapse series of DMSO-treated ControlcKO, 4 µM Yoda1-treated ControlcKO, DMSO-treated Piezo1-cKO, ControlGoF, and Piezo1-GoF keratinocytes. Brackets separate Piezo1-cKO and Piezo1-GoF background cell types. Color of cell boundary outline indicates passage of time. Scale bar = 25 µm. (D) Violin plots showing the instantaneous protrusion (positive) and retraction (negative) velocities at each position of the segmented cell edges for each frame of time-lapse series videos. Plot shows the combined data from six DMSO and Yoda1 treated ControlcKO cells, respectively, nine Piezo1-cKO cells, five ControlGoF, and six Piezo1-GoF cells from two independent experiments each. p Values calculated using Mann-Whitney test; d values show Cohen’s d which is calculated as test condition minus the respective control condition. (E) Representative heatmaps corresponding to the cells shown in C illustrating cell edge velocities along segmented cell boundaries from time-lapse series. See also Figure 5—figure supplement 2. (F, G, H) Representative DIC kymograph taken at the wound edge and kymograph of the binarized version of the same video, where the cell monolayer is represented in black and cell-free space of the wounded area in white. Kymographs illustrate wound edge dynamics during an in vitro scratch assay performed in (F) control (DMSO)-treated monolayers, (G) control scratches treated with 4 μM Yoda1, and (H) Piezo1-cKO monolayers (right pair). Scale bar = 20 μm (vertical) and 100 min (horizontal). The data in F, G are representative four independent experiments from keratinocytes from three biological repeats for each condition. The data in H are representative of three independent experiments using keratinocytes from three biological repeats. See also Figure 5—figure supplements 3–4 and Figure 5—videos 5–7.
-
Figure 5—source code 1
Code used to analyze data shown in Figure 5.
See also Code availability.
- https://cdn.elifesciences.org/articles/65415/elife-65415-fig5-code1-v2.zip
-
Figure 5—source data 1
PIEZO1 activity promotes cellular retraction and increases edge velocity.
(Sheet 1) X,Y coordinates to create representative overlays of cell boundary outlines in Figure 5C detected and segmented from differential interference contrast (DIC) time-lapse series of DMSO-treated ControlcKO, 4 µM Yoda1-treated ControlcKO, DMSO-treated Piezo1-cKO, ControlGoF, and Piezo1-GoF keratinocytes. This dataset is available on Dryad.
- https://cdn.elifesciences.org/articles/65415/elife-65415-fig5-data1-v2.xlsx
-
Figure 5—source data 2
PIEZO1 activity promotes cellular retraction and increases edge velocity.
(Sheet 1) Data used to create violin plots in Figure 5D showing the instantaneous protrusion (positive) and retraction (negative) velocities at each position of the segmented cell edges for each frame of time-lapse series videos. This dataset is available on Dryad.
- https://cdn.elifesciences.org/articles/65415/elife-65415-fig5-data2-v2.xlsx
-
Figure 5—source data 3
PIEZO1 activity promotes cellular retraction and increases edge velocity.
(Sheets 1–5) Data used to create representative heatmaps corresponding to the cells shown in Figure 5E illustrating cell edge velocities along segmented cell boundaries from time-lapse series.
- https://cdn.elifesciences.org/articles/65415/elife-65415-fig5-data3-v2.xlsx
Kymograph-based quantitation is limited to one point along the cell edge. To more objectively investigate the effect that PIEZO1 activation has on cell morphodynamics, cells were segmented for each frame of a DIC time-lapse series for the following conditions: ControlcKO cells before and after Yoda1 addition, Piezo1-cKO cells, ControlGoF cells, and Piezo1-GoF keratinocytes (Figure 5C). By comparing segmented outlines between frames, we could obtain the velocity of the cell edge at every position along the detected boundaries for protrusion events (positive velocities) and retraction events (negative velocities). Yoda1 treatment resulted in a significant increase in edge velocities compared to DMSO-treated ControlcKO cells (Figure 5D). Yoda1, which is expected to globally activate PIEZO1 channels, resulted in an increase of cell edge velocity during both protrusion and retraction events, though the increase in retraction velocity was greater. In contrast, Piezo1-cKO keratinocytes showed a reduction in edge velocities relative to littermate ControlcKO cells. Heatmaps of cell edge velocity illustrate the robustness of this response (Figure 5E). Consistent with our observations of Yoda1 treatment, Piezo1-GoF keratinocytes also showed a significant increase in edge velocities relative to littermate ControlGoF (Figure 5D, Figure 5—figure supplement 2). Interestingly, there was a clear increase in the proportion of retracting positions relative to protruding positions in GoF keratinocytes. These results reveal that PIEZO1 activity regulates cell edge dynamics and further support our observations that PIEZO1 activity increases cellular retraction.
We then asked whether PIEZO1-mediated retraction events observed in single cells are relevant in the context of a wounded cell monolayer. We generated scratch wounds in keratinocyte monolayers and performed DIC time-lapse imaging of the healing monolayer in the presence and absence of 4 μM Yoda1. Monolayers of control keratinocytes advanced forward into the cell-free space under DMSO-treated control conditions (Figure 5F, Figure 5—video 5), while the presence of 4 μM Yoda1 increased retraction events which prevented the monolayer from advancing far into the wound bed (Figure 5G, Figure 5—video 5). Remarkably, in 35% of fields of view monitored in scratch assays we observed that Yoda1 treatment resulted in an increase in scratch area instead of wound closure (Figure 1L). In Piezo1-cKO monolayers we observed cells protrude forward into the cell-free space to close scratch wounds to a greater extent than controls (Figure 5H, Figure 5—video 6), while GoF monolayers did so to a lower extent (Figure 5—figure supplement 3, Figure 5—video 7). Additionally, no effect of Yoda1 addition was seen on the rate of advancement in kymographs taken at the wound edge of Piezo1-cKO monolayers (Figure 5—figure supplement 4). Collectively, our results show that PIEZO1 activity increases cellular retraction in keratinocytes, both in single cells and monolayers, which has a net effect on cell migration.
Taken together, our results demonstrate that PIEZO1 induces cellular retraction to slow single and collective cell migration and thus causes delayed wound healing. We propose that dynamic enrichment of the channel protein serves to locally amplify channel activity and the downstream retraction events. In wound healing monolayers of keratinocytes, PIEZO1 enrichment and the subsequent wound edge retraction provide a molecular mechanism for how PIEZO1 slows wound healing, while absence of the channel accelerates wound healing.
Discussion
Our findings demonstrate that epidermal-specific Piezo1 knockout resulted in faster wound healing in mice, and conversely a Piezo1-GoF mutation slowed wound healing. We recapitulate this observation in vitro, and show through a combination of orthogonal assays in single cells and in monolayers that PIEZO1 activity modulated by dynamic spatial reorganization of the channel protein slows keratinocyte cell migration during re-epithelialization. These findings provide physiological evidence for the role of a mechanically activated ion channel in wound healing and suggest potential therapeutics through a targeted inhibition of PIEZO1, perhaps applied topically, that could help speed wound healing, potentially reducing risk of infection. However, further in-depth analysis regarding the quality of wound healing following PIEZO1 inhibition is required. Given that speedy wound healing affords an evolutionary advantage and that PIEZO1 activity slows wound healing, a puzzling question arises regarding the role of PIEZO1 expression in keratinocytes. Perhaps, there is an advantage to slower healing in the presence of PIEZO1, or the channel is important for other functions in keratinocytes. Consistent with the latter idea, a recent study by Moehring et al. reports that keratinocyte PIEZO1 is critical for sensory afferent firing and behavioral responses to innocuous and noxious mechanical stimulation (Moehring et al., 2020). As such, it would also be important to determine that inhibiting Piezo1 to speed wound healing does not have detrimental effects on normal mechanosensation.
Ca2+ signals control many aspects of cell migration, including lamellipodial dynamics, traction force generation, rear retraction, focal adhesion turnover, and migration directionality (Wei et al., 2012; Tsai et al., 2015; Canales et al., 2019). While mechanically activated ion channels were proposed to contribute to Ca2+ signaling in single cell migration in vitro as early as 20 years ago (Lee et al., 1999; Doyle et al., 2004; Wei et al., 2009; Tsai and Meyer, 2012; Patkunarajah et al., 2020), many important questions have remained unanswered, including those related to channel identity, functional effects in collective cell migration, and physiological contribution during wound healing. Using chemical activation as well as genetic modulation of PIEZO1, we provide evidence for its involvement in regulating cellular retraction events in single cells as well as in collective cell migration during keratinocyte re-epithelialization.
Notably, the effects we observed on scratch wound closure and cell edge retraction speeds following Yoda1 activation of PIEZO1 were consistently larger than the effects of Piezo1-GoF mutation. This is not surprising as the GoF mutation increases ion flux through PIEZO1 channels, without significantly affecting channel activation (Glogowska et al., 2017; Bae et al., 2013). Thus, channel activation in GoF keratinocytes is expected to occur based on subcellular localization of PIEZO1 and of the cellular forces that activate them. In contrast, Yoda1 treatment would globally activate PIEZO1 channels in the plasma membrane, leading to a larger effect.
One of the most surprising findings to emerge from our studies is the highly dynamic nature of the spatial localization of PIEZO1 channels in migrating cells. Based on this finding, we propose a novel mechanism regulating cell migration wherein spatiotemporal enrichment of PIEZO1 channels serves to localize and amplify channel activity, and regulate contractile forces, to spatially control cellular retraction events. Piezo1 has been implicated in cell migration in different cell types in vitro (Maneshi et al., 2018; McHugh et al., 2012; Chubinskiy-Nadezhdin et al., 2019; Hung et al., 2016; Li et al., 2015; Yu et al., 2020); however, reports of the effect of the channel on migration have varied in the literature, with channel activity supporting migration in some cell types and inhibiting migration in others. Perhaps, a determining factor of the channel’s impact on cell migration is how spatiotemporal localization of the channel is regulated in a given cell type.
Our observations spark several new questions regarding the regulation and functional impacts of PIEZO1’s localization and clustering dynamics. Interestingly, molecular dynamics simulations of PIEZO1 suggest that interactions between neighboring PIEZO1 channels may enable cooperative gating between channels (Jiang et al., 2021). Clustering of the bacterial mechanosensitive channel MscL has also been reported, but computational simulations predict these clusters in fact decrease channel open probability, providing a defence against unwanted channel gating that may cause osmotic shock (Paraschiv et al., 2020; Grage et al., 2011). Experimental evidence for functional interaction between PIEZO1 channels remains pending and two recent patch clamp studies examining this question come to divergent conclusions (Lewis and Grandl, 2021; Wijerathne et al., 2021).
It is well established that retraction during cell migration occurs due to force generated by myosin II (Cramer, 2013; Ridley, 2011; Aguilar-Cuenca et al., 2014), and we previously showed that myosin II-mediated cellular traction forces elicit localized Piezo1 Ca2+ flickers (Ellefsen et al., 2019). Since myosin II activation is enhanced by intracellular Ca2+ (Somlyo and Somlyo, 2003), we speculate that Piezo1 may induce cellular retraction through a feedforward loop between Piezo1 and myosin II: traction force generation by myosin II cause Piezo1-mediated Ca2+ influx, which in turn may increase myosin II phosphorylation and force generation through the Ca2+-regulated Myosin Light Chain Kinase. Enrichment of Piezo1 in subcellular regions would amplify this effect and result in a localized retraction. Supporting this model, Piezo1-mediated Ca2+ events were recently found to elicit retraction of developing endothelial tip cells during vascular pathfinding (Liu et al., 2020).
Efficient migration requires protrusion of the cell lamellipodia which is stabilized via the formation of focal adhesions. Without stabilization, lamellipodia protrusions retract backward causing membrane ruffling and reducing migration speed (Borm et al., 2005). We observe an increase in cell edge velocity in keratinocytes with increased PIEZO1 activity (in both Piezo1-GoF and Yoda1-treated cells) indicating that PIEZO1-mediated effects on cell edge velocity may be the predominant mechanism contributing to inefficient migration. An intriguing paradox observed in our data is that both Piezo1-cKO and Piezo1-GoF keratinocytes exhibit straighter migration trajectories. We also observe that PIEZO1 localization and increased activity appears linked to cell polarization; thus one possible explanation that warrants further study is that the GoF mutation stabilizes PIEZO1 clusters so that once the channels localize to the rear of the cell, the cell retains its polarization and migration directionality.
Cell migration involves a complex orchestration of events, including sub-cellular dynamics in which cytoskeletal processes in different compartments of the cell need to be implemented in a precise spatiotemporal order. How this is achieved remains an open question. Our findings suggest that spatiotemporal enrichment dynamics of Piezo1 play a role in this coordination. More broadly, our findings provide a mechanism by which nanoscale spatial dynamics of Piezo1 channels can control tissue-scale events, a finding with implications beyond wound healing to processes as diverse as development, homeostasis, disease, and repair.
Materials and methods
Reagent type (species) or resource | Designation | Source or reference | Identifiers | Additional information |
---|---|---|---|---|
Genetic reagent(mouse) | Krt14Cre;Piezo1fl/fl(Piezo1-cKO) | This paper | Generated by breeding Piezo1fl/fl mice (Jax stock 029213) with K14Cre mice(The Jackson Laboratory, stock 004782) | |
Genetic reagent(mouse) | Krt14Cre;Piezo1cx/+and Krt14Cre;Piezo1cx/cx(Piezo1-GoF) | This paper | Generated by breeding Piezo1cx/cx mice (Ma et al., 2018) with K14Cre mice. | |
Genetic reagent(mouse) | Piezo1-tdTomato | JAX; Ranade et al., 2014 | 029214(RRID:IMSR_JAX:029214) | |
Genetic reagent(mouse) | Piezo1 LacZ reporter mice | JAX | 026948(RRID:IMSR_JAX:026948) | |
Biological sample (mouse) | Murine keratinocytes | UC Irvine,The Scripps Research Institute | Freshly isolated from P0–P5 mouse pups | |
Antibody | anti-Keratin 14 (Rabbit polyclonal) | Covance | Cat#PRB-155P(RRID:AB_292096) | IF (1:1000) |
Antibody | anti-Keratin K10 (Rabbit polyclonal) | Covance | Cat#PRB-159P(RRID:AB_291580) | IF (1:1000) |
Antibody | anti-Rabbit Alexa Fluor 488 (Goat polyclonal) | Invitrogen | Cat#A11008(RRID:AB_143165) | IF (1:1000) |
Sequence-based reagent | Piezo1 | Thermo Fisher | PCR primersTaqman Assay ID: Mm01241570_g1 | |
Sequence-based reagent | Piezo2 | Thermo Fisher | PCR primersTaqman Assay ID: Mm01262433_m1 | |
Sequence-based reagent | Gapdh | Thermo Fisher | PCR primersTaqman Assay ID: Mm99999915_g1 | |
Sequence-based reagent | Krt14 | Thermo Fisher | PCR primersTaqman Assay ID: Mm00516876_m1 | |
Commercial assay or kit | SuperScript III | Invitrogen (Thermo Fisher) | Cat#12574026 | Synthesizing cDNA |
Commercial assay or kit | RNeasy kit | Qiagen | Isolating RNA | |
Chemical compound, drug | Cal-520 AM | AAT Bioquest Inc | Cat#21130 | |
Chemical compound, drug | Yoda1 | TOCRIS | 558610 | |
Software, algorithm | Origin Pro | Originlab | OriginPro(RRID:SCR_014212) | |
Software, algorithm | VAMPIRE | Phillip et al., 2021 | Version 1.0 (RRID: SCR_021721) | https://github.com/kukionfr/VAMPIRE_open |
Software, algorithm | ADAPT | Barry et al., 2015 | (RRID: SCR_006769) | https://github.com/djpbarry/Adapt |
Software, algorithm | DiPER | Gorelik and Gautreau, 2014 | (RRID:SCR_021720) | |
Software, algorithm | Flika | Ellefsen et al., 2019 | Version 0.2.17 (RRID: SCR_021719) | https://flika-org.github.io/ https://github.com/kyleellefsen/detect_puffs |
Software, algorithm | Cell Tracker | Piccinini et al., 2016 | Version 1.1 (RRID:SCR_021718) | http://celltracker.website/index.html |
Software, algorithm | Cellpose | Stringer et al., 2021 | Version 0.06 (RRID:SCR_021716) | https://github.com/MouseLand/cellpose |
Software, algorithm | ilastik | Berg et al., 2019 | Version 1.4.0b13 (RRID:SCR_015246) | https://www.ilastik.org/ |
Software, algorithm | Wolfram Mathematica | Wolfram | Wolfram Mathematica 12 | |
Software, algorithm | FIJI | Schindelin et al., 2012 | Version 1.53 c(RRID:SCR_002285) | https://imagej.net/software/fiji/ |
Software, algorithm | Python | https://www.python.org/ | Version 3.7.0(RRID:SCR_008394) | |
Software, algorithm | Kymobutler | Jakobs et al., 2019 | Version: V1v0v2.wl (RRID:SCR_021717) | https://github.com/alexlib/KymoButler-1 |
Other | DAPI stain | Invitrogen | D1306(RRID:AB_2629482) | 1:50,000 |
Animals
All studies were approved by the Institutional Animal Care and Use Committee of University of California at Irvine and The Scripps Research Institute, as appropriate, and performed in accordance with their guidelines. Piezo1 LacZ reporter mice (JAX stock 026948) and Piezo1-tdTomato reporter mice, expressing a C-terminal fusion of Piezo1 with tdTomato (Piezo1-tdTomato; JAX stock 029214), were generated in a previous study (Ranade et al., 2014). Skin-specific Piezo1-cKO mice were generated by breeding Piezo1fl/fl mice (Cahalan et al., 2015) (Jax stock 029213) with K14Cre (The Jackson Laboratory, stock 004782). Skin-specific Piezo1-GoF mice were generated by breeding mice with conditional GoF Piezo1 allelle (Piezo1cx/cx mice [Ma et al., 2018]) with K14Cre mice. Piezo1fl/fl mice were generated in C57BL/6 background and Piezo1cx/cx mice were initially generated in BALB/c background and then maintained in C57BL/6 for >10 generations. K14Cre mice were in the C57BL/6 background.
Keratinocyte isolation
Request a detailed protocolP0–P5 mice were anesthetized with ice prior to decapitation. Bodies were placed in 10% povidone for 1 min, rinsed with sterile PBS, prior to soaking in 70% ethanol for a further minute, and rinsed again with sterile PBS. Subsequently, the entire upper dorsal skin above the abdomen was separated from the body. Dorsal skin was left to dissociate in either 0.25% trypsin/EDTA (Gibco) for 1 hr at 37°C or 1× dispase solution (CellnTec CnT-DNP-10) at 4°C overnight for 15–18 hr. After incubation, the epidermis was gently separated from the dermis, laid flat, dorsal side down in Accutase (CellnTec CnT-Accutase-100) and incubated for 30 min at room temperature. The epidermis was then transferred to a dish of either CnT-02 or CnT-Pr media (CellnTec), supplemented with 10% FBS and 1% penicillin/streptomycin. The epidermis was cut into small pieces with scissors prior to agitation on a stir plate for 30 min. Cells were then filtered through a 70 µm cell strainer (Falcon) and spun down at 1200 rpm for 5 min. The pellet was resuspended in CnT-Pr media (CellnTec) supplemented with ISO-50 (1:1000) (CellnTec) and Gentamicin (50 µg/ml) (Thermo Fisher), prior to counting and plating.
Keratinocyte culture
Request a detailed protocolFor live-cell imaging, primary keratinocytes were plated on #1.5 glass-bottom dishes (Mat-Tek Corporation) coated with 10 µg/ml fibronectin (Fisher Scientific, CB-40008A). For monolayer experiments, cells were plated at 1.5 × 105 cells/dish in the 14 mm glass region of dishes. For sparse cell migration experiments and for Ca2+ imaging experiments, cells were plated at 1.5 × 104 cells/dish in the 14 mm glass region of dishes. Keratinocytes were imaged following at least 2 days in Cnt-Pr-D (CellnTec) differentiation media.
Real-time quantitative PCR
Request a detailed protocolAfter initial keratinocyte isolation and filtering through a 70 µm cell strainer, cells were filtered again through a 40 µm strainer. The filtered solution was spun down and a cell pellet was obtained for RNA isolation. Total RNA was isolated using the RNeasy kit (Qiagen), following which cDNA was synthesized using Superscript III (Invitrogen) and was used for subsequent qPCR experiments (ABI 7900HT fast real-time system). qPCR Taqman probes (Thermo Fisher) used were Piezo1: Assay ID Mm01241570_g1; Piezo2; Assay ID Mm01262433_m1, Krt14; Assay ID Mm00516876_m1 and Gapdh; Assay ID Mm99999915_g1. PCR reactions were run in triplicate.
X-Gal/LacZ staining
Request a detailed protocolDorsal skin was harvested as described above, cryopreserved in OCT, and sectioned into 8 µm thick slices. Skin cryosections were allowed to completely dry prior to being fixed in ‘fix buffer’ composed of 1× PBS, 5 mM EGTA (Sigma Cat#E4378), 2 mM MgCl2, 0.2% glutaraldehyde (Sigma Cat#G-7776), pH 7.4 for 15 min at room temperature. Next they were washed with ‘wash buffer’ composed of 1× PBS, 2 mM MgCl2 twice for 5 min each. X-gal staining buffer composed of 1× PBS, 2 mM MgCl2, 5 mM potassium ferrocyanide [K4Fe(CN)6·3H20] (Sigma Cat# P-9287), 5 mM potassium ferricyanide [K3Fe(CN)6] (Sigma Cat#P-8131), and 1 mg/ml X-gal [5-bromo-4-chloro-3-indolyl-β-D-galactoside] was made fresh. Then, tissue slides were incubated overnight at 37°C in the ‘X-gal staining buffer’ inside a humidified chamber. The following day, slides were rinsed with 1× PBS and counterstained with Nuclear Fast Red. Slides were then fixed with 4% PFA for longer preservation.
Immunofluorescence staining
Request a detailed protocolFor immunostaining of skin sections in Figure 1—figure supplement 1, dorsal skin was prepared and sectioned as for X-Gal staining. Skin cryosections were fixed for 10 min in cold acetone, washed twice in 1× PBS prior to blocking for 30 min in 10% normal goat serum at room temperature. Primary antibodies used were Rabbit anti-Keratin 14 (Covance, Cat#PRB-155P), 1:1000 (1 µg/ml), and Rabbit anti-Keratin 10 (Covance, Cat#PRB-159P), 1:1000 (1 µg/ml). Secondary antibody used was Goat anti-Rabbit Alexa Fluor 488 (Invitrogen, Cat#A11008), 1:1000. Nuclei were stained by DAPI (Invitrogen, Cat#D1306), 1:50,000. All antibody incubations were performed at room temperature, for 1 hr in 1% BSA in PBS. Slides were mounted in gelvatol containing DAPI.
Microscopy and image analysis
Microscopy
Request a detailed protocolUnless otherwise stated, in vitro images were taken using an Olympus IX83-ZDC microscope, equipped with an automated four-line cellTIRF illuminator. A full enclosure environmental chamber (Tokai Hit) allowed cells to be imaged at 37°C with 5% CO2 ensuring optimal cell health during time-lapse experiments. Stage movement was controlled by a programmable motorized stage (ASI) while an Olympus ZDC autofocus control unit allowed for samples to remain in focus throughout imaging periods. The open-source microscopy software μManager was used to control the microscope and acquire images for all except Figures 1A, I, 5A and Figure 1—figure supplement 1. Images for Figures 1D,G,4, 5, Figure 3A, Figure 4, Figure 5B, Figure 4—figure supplement 1, Figure 5—figure supplement 1, and Figure 1—videos 1 and 2, Figure 3—video 1, Figure 4—videos 1–3–, Figure 5—video 2; Figure 5—video 3; Figure 5—video 4 were taken using a PLAPO 60× oil immersion objective with a numerical aperture of 1.45. Images for Figure 5A, Figure 5—video 1 were taken using a UPlanSApo 40× dry objective with a numerical aperture of 0.95. Images taken for 1,2,51,2,5Figure 1K, Figure 2A, Figure 3, Figure 5F, G, H, Figure 5—figure supplement 3, and Figure 2—videos 1 and 2, Figure 5—video 5 were taken using a UPlanSApo 10× dry objective with a numerical aperture of 0.40. Images for Figures 1D, G, K, 2, 5, all figure supplements except Figure 1—figure supplement 1 and all videos were acquired using a Hamamatsu Flash 4.0 v2+ scientific CMOS camera. Images for Figure 1—figure supplement 1B were taken using a Hamamatsu C4742-95-12ER Digital CCD camera.
Imaging Piezo1 Ca2+ flickers (Figure 1C-H, Figure 1—videos 1 and 2)
Request a detailed protocolAs described previously (Ellefsen et al., 2019), TIRF microscopy was used for the detection of Ca2+ flickers. Keratinocytes were loaded through the incubation of 2 μM Cal-520 AM (AAT Bioquest Inc) with 0.04% Pluronic F-127 (Thermo Fisher) in phenol red-free DMEM-F12 (Cat#11039047, Gibco) for 30–35 min at 37 °C, washed three times, and incubated at room temperature for 10–15 min prior to imaging. Cells were imaged at room temperature in a bath solution comprising 148 mM NaCl, 3 mM KCl, 3 mM CaCl2, 2 mM MgCl2, 8 mM glucose, and 10 mM HEPES (pH adjusted to 7.3 with NaOH, osmolarity adjusted to 313 mOsm/kg with sucrose). Cal-520 fluorescence was elicited by excitation with a 488 nm laser line and images were acquired at a frame rate of 9.54 frames/s using a Hamamatsu Flash 4.0 v2+ scientific CMOS camera.
Piezo1 Ca2+ flicker analysis (Figure 1C-H, Figure 1—videos 1 and 2)
Request a detailed protocolCa2+ flickers were automatically detected as previously described (Ellefsen et al., 2019) using the detect_puffs plugin (https://github.com/kyleellefsen/detect_puffs) for the open-source image processing program, Flika (https://flika-org.github.io/). This plugin was used to identify and localize flicker events in recorded videos. Each video is a microscope field of view which contains one or more keratinocytes. To normalize any potential variability in cell number or size between samples, flicker frequency by cell area was computed for each field of view. Cell area was measured by using the FIJI (Schindelin et al., 2012) plugin SIOX: Simple Interactive Object Extraction to create binary masks and compute cell area.
In vitro wound healing assay (Figure 1L, Figure 1—figure supplement 3)
Request a detailed protocolPrimary keratinocytes were either densely plated onto Mat-Tek dishes or seeded into a two-well silicone insert in a 35 mm dish (ibidi, 81176). Cells were cultured until monolayer confluence was reached. Subsequently, scratch wounds were generated by either scratching monolayers with a 10 µl pipette (for cells plated on Mat-Tek dishes) or by removing the barrier insert from the dish to create a 500 µm cell-free gap (for cells in ibidi dishes). The dishes were washed with cell culture medium to remove floating cells and cell debris. For pharmacology experiments, Yoda1 or equivalent concentration of DMSO control was added into the dishes immediately prior to imaging. Dishes were imaged by either DIC or phase-contrast microscopy at 37°C (5% CO2) for indicated time points.
Single cell tracking assay (Figure 2, Figure 2—videos 1 and 2; Figure 2—figure supplements 1–3)
Request a detailed protocolPrimary keratinocytes sparsely seeded on fibronectin-coated glass-bottom dishes were allowed to migrate freely for up to 16.67 hr at 37°C with 5% CO2 in bath solution composed of Cnt-Pr-D (CellnTec) culture media with extracellular Ca2+ concentration adjusted to 1.2 mM. Time-lapse DIC images at multiple microscope fields of view were acquired in each dish of cells at 5 min intervals for the imaging period. When collecting trajectories we only considered cells which (1) stay within the field of view during the imaging period and (2) did not come into contact with other keratinocytes. The center of the cell body was the tracked position of the cell. The initial positions of cells were manually identified, after which the positions of migrating cells were automatically tracked using the Cell Tracker software (https://celltracker.website/index.html) (Piccinini et al., 2016). Cell trajectories were logged and exported into Microsoft Excel. Further analysis was subsequently performed using the published open-source algorithm, DiPer (Gorelik and Gautreau, 2014) to obtain average instantaneous speed, MSD, directionality analysis, and trajectory flower plots.
VAMPIRE shape mode analysis (Figure 3B-G)
Request a detailed protocolFor analyses of cellular morphometrics, time-lapse images from single cell tracking assays were manually segmented using the generalist deep learning-based segmentation algorithm, Cellpose (https://github.com/MouseLand/cellpose) (Stringer et al., 2021). Segmented outputs from Cellpose were manually refined using FIJI to ensure the accuracy of detected shapes before being fed into the VAMPIRE algorithm (Phillip et al., 2021) (https://github.com/kukionfr/VAMPIRE_open). VAMPIRE allows the profiling and classification of cells into shape modes based on equidistant points along cell contours. The number of coordinates to extract cell contours was set to 400 to ensure accurate representation of cell shapes and 20 shape modes were used for the dataset.
PIEZO1-tdTomato time-lapse imaging (Figures 3A and 4, Figure 4—figure supplement 1, Figure 3—video 1, Figure 4—videos 1–3)
Request a detailed protocolPrimary PIEZO1-tdTomato keratinocytes were cultured either sparsely or as confluent monolayers. Monolayers were scratched using a 10 µl pipette tip immediately prior to imaging. Experiments were performed in Cnt-Pr-D (CellnTec) culture media with added 1.2 mM Ca2+. Either single cells or, for monolayer scratch experiments, regions along the initial wound edge were marked using a programmable stage and imaged throughout the imaging period as cells migrated to close the wound. PIEZO1-tdTomato channels were illuminated using a 561 nm laser and imaged using TIRF microscopy. TIRF and DIC snapshots at regions of interest were sequentially acquired every 10 (Figures 3A and 5B and Figure 3—video 1, Figure 4—video 1) or 30 min (Figure 5A, C and F, Figure 4—figure supplement 1, Figure 4—videos 2 and 3) over the course of up to 16.67 hr. To increase the signal-to-background ratio, time-lapse images were processed from the original recording by subtracting every frame of a movie by (1) the median value z-projection image and then (2) the minimum value z-projection image. The FIJI (Schindelin et al., 2012) plugin KymoResliceWide was then used to construct kymographs by taking the average intensity of the transverse of a 100 pixel wide line drawn at ROIs along the wound edge. ROIs were chosen so that they would capture the wound edge for the entire duration it was present within the microscope field of view. At least one kymograph was generated from each unique microscope field of view. Impartial identification and tracking of periods of channel enrichment was performed using the Kymobutler tool in Wolfram Mathematica (https://gitlab.com/deepmirror/kymobutler) (Jakobs et al., 2019). Only puncta located at the wound edge that were successfully tracked for at least 70 min were collected for further analyses. The pixel classification function of the computer vision software, Ilastik (https://www.ilastik.org/) (Berg et al., 2019), was used to classify DIC images and create binarized movies used for generating binarized kymographs in Figure 5D and E.
Migration dynamics assay (Figure 5A-B, Figure 5—figure supplement 1A; Figure 5—videos 1–4)
Request a detailed protocolCells were imaged via DIC microscopy at 37°C with 5% CO2, with snapshots taken at 5 s intervals for at least 30 min in Cnt-Pr-D culture media (CellnTec) with added 1.2 mM Ca2+ and 0.0004% DMSO. After acquiring baseline images, the media was changed and 4 µM Yoda1 was added to the bath solution. After allowing the drug to act for 5–7 min, imaging was resumed for at least 55 min. Experiments were performed multiple times on independent experiment days. Kymographs were created from representative cells using the FIJI (Schindelin et al., 2012) plugin KymoResliceWide (https://imagej.net/KymoResliceWide). Kymographs were built by taking one pixel width lines at ROIs along the cell’s leading edge.
ADAPT analysis of keratinocyte morphodynamics (Figure 5C-E, Figure 5—figure supplement Figure 5—figure supplements 1C and 2)
Request a detailed protocolDIC time-lapse images from migration dynamics time-lapse series were binarized using the pixel classification function of the computer vision software, Ilastik (https://www.ilastik.org/) (Berg et al., 2019). Binarized images were then analyzed using the FIJI (Schindelin et al., 2012) plugin ADAPT (https://github.com/djpbarry/Adapt) (Barry et al., 2015) in order to create cell boundary outline overlays and determine the velocity at each position along the cell edge. A Python script used to transform velocity map ADAPT outputs into matrices which were then used to generate heatmaps and velocity violin plots.
Wound edge dynamics assay (Figure 5F-H, Figure 5—figure supplements 3 and 4, Figure 5—videos 5–7)
Request a detailed protocolSimilar to the in vitro wound healing assay described above, primary keratinocytes were densely seeded and cultured to form monolayers. Scratch wounds were generated by scratching monolayers with a 10 µl pipette tip immediately prior to imaging. Dishes were washed 3× with cell culture media to remove cell debris. Yoda1 (4 µM) or equivalent concentration of DMSO control was added to the bath media immediately prior to imaging. DIC snapshots were taken every 5 min at ROIs along the wound edge for at least 9 hr. Representative kymographs were created using one pixel-wide ROIs using KymoResliceWide. Binarized movies generated by using the FIJI (Schindelin et al., 2012) plugin Trainable Weka Segmentation (Arganda-Carreras et al., 2017) (https://imagej.net/Trainable_Weka_Segmentation) were used to create binarized kymographs.
In vivo wound healing assay
Request a detailed protocolAdult (3–4 months) male and female mice were anesthetized with isoflurane and placed on a heated blanket. The dorsal hair was shaved and further removed by hair-removal cream. Two full-thickness wounds were created in the upper dorsal skin above the abdomen using a 4 mm wide dermal biopsy punch (Integra LifeSciences Corporation). Wounded areas were patched with medical dressing, Tegaderm (3 M). Wound sizes were measured with a scale loupe (Peak Optics, #1975) at day 6 to compare healing progress. Both the short (dS) and long (dL) diameters of the oval-shaped wounds were measured and used to calculate an overall wound area using the equation: dS × dL × π.
Statistical analysis
Request a detailed protocolSample sizes are indicated in corresponding figures. Cumming estimation plots were generated and Cohen’s d in all plots (except Figure 5D) was calculated using an online estimation stats tool (https://www.estimationstats.com) (Ho et al., 2019). Estimation plots show the raw data plotted on the upper axes with bars beside each group denoting the sample mean ± s.d.; the mean difference and Cohen’s d effect size is plotted on the lower axes. On the lower plot, the mean difference is depicted as a dot; the 95% confidence interval is indicated by the ends of the bold vertical error bar. OriginPro 2020 (OriginLab Corporation) was used for calculating p values (for all figures except for Figure 5D) and generating plots used in Figure 1B, Figure 2B, C, D, Figure 3D, E, F, G, Figure 4G, Figure 5D1—5, Figure 1—figure supplement 2, and Figure 2—figure supplements 1–3. All plots generated in OriginLab are presented as the mean ± SEM. Statistical tests used to calculate p values are indicated in figure legends. For Figure 5D, a Python script was used for calculating Cohen’s d and p values.
Data availability
Request a detailed protocolThe datasets plotted in Figure 1E,H,J,L, Figure 2B, C, D, E, Figure 3D, E, F, G, Figure 4G, Figure 5E, Figure 1—figure supplements 2–3, Figure 2—figure supplements 1–3, and Figure 5—figure supplements 1C and 2 have been uploaded as source data files. Source data files for Figure 5C and D have been uploaded to Dryad (doi:10.5061/dryad.hdr7sqvjr).
Code availability
Request a detailed protocolData availability
The datasets for graphs included in each figure have been made available as source data files. Source data files for Figure 5C and 5D have been uploaded to Dryad (https://doi.org/10.5061/dryad.hdr7sqvjr).
-
Dryad Digital RepositorySpatiotemporal dynamics of PIEZO1 localization controls keratinocyte migration during wound healing.https://doi.org/10.5061/dryad.hdr7sqvjr
References
-
Myosin II in mechanotransduction: master and commander of cell migration, morphogenesis, and cancerCellular and Molecular Life Sciences 71:479–492.https://doi.org/10.1007/s00018-013-1439-5
-
Open source software for quantification of cell migration, protrusions, and fluorescence intensitiesThe Journal of Cell Biology 209:163–180.https://doi.org/10.1083/jcb.201501081
-
ilastik: interactive machine learning for (bio)image analysisNature Methods 16:1226–1232.https://doi.org/10.1038/s41592-019-0582-9
-
Forces driving epithelial wound healingNature Physics 10:683–690.https://doi.org/10.1038/nphys3040
-
Agonist-induced Piezo1 activation suppresses migration of transformed fibroblastsBiochemical and Biophysical Research Communications 514:173–179.https://doi.org/10.1016/j.bbrc.2019.04.139
-
Mechanism of cell rear retraction in migrating cellsCurrent Opinion in Cell Biology 25:591–599.https://doi.org/10.1016/j.ceb.2013.05.001
-
Calcium transients induce spatially coordinated increases in traction force during the movement of fish keratocytesJournal of Cell Science 117:2203–2214.https://doi.org/10.1242/jcs.01087
-
Epithelial mechanobiology, skin wound healing, and the stem cell nicheJournal of the Mechanical Behavior of Biomedical Materials 28:397–409.https://doi.org/10.1016/j.jmbbm.2013.04.023
-
Skin: histology and physiology of wound healingFacial Plastic Surgery Clinics of North America 19:441–453.https://doi.org/10.1016/j.fsc.2011.06.009
-
Bilayer-mediated clustering and functional interaction of MscL channelsBiophysical Journal 100:1252–1260.https://doi.org/10.1016/j.bpj.2011.01.023
-
Mechanobiology of collective cell behavioursNature Reviews. Molecular Cell Biology 18:743–757.https://doi.org/10.1038/nrm.2017.98
-
Piezos thrive under pressure: mechanically activated ion channels in health and diseaseNature Reviews. Molecular Cell Biology 18:771–783.https://doi.org/10.1038/nrm.2017.92
-
How cells channel their stress: Interplay between Piezo1 and the cytoskeletonSeminars in Cell & Developmental Biology 71:3–12.https://doi.org/10.1016/j.semcdb.2017.06.018
-
Dynamic Clustering Regulates Activity of Mechanosensitive Membrane ChannelsPhysical Review Letters 124:048102.https://doi.org/10.1103/PhysRevLett.124.048102
-
Random versus directionally persistent cell migrationNature Reviews. Molecular Cell Biology 10:538–549.https://doi.org/10.1038/nrm2729
-
CellTracker (not only) for dummiesBioinformatics 32:955–957.https://doi.org/10.1093/bioinformatics/btv686
-
Mechanoregulation of Wound Healing and Skin HomeostasisBioMed Research International 2016:3943481.https://doi.org/10.1155/2016/3943481
-
Fiji: an open-source platform for biological-image analysisNature Methods 9:676–682.https://doi.org/10.1038/nmeth.2019
-
Cellpose: a generalist algorithm for cellular segmentationNature Methods 18:100–106.https://doi.org/10.1038/s41592-020-01018-x
-
CA2+ signaling in cytoskeletal reorganization, cell migration, and cancer metastasisBioMed Research International 2015:409245.https://doi.org/10.1155/2015/409245
-
Calcium gradients underlying cell migrationCurrent Opinion in Cell Biology 24:254–261.https://doi.org/10.1016/j.ceb.2011.12.002
-
Actin-myosin network reorganization breaks symmetry at the cell rear to spontaneously initiate polarized cell motilityThe Journal of Cell Biology 178:1207–1221.https://doi.org/10.1083/jcb.200706012
-
Piezo1 regulates migration and invasion of breast cancer cells via modulating cell mechanobiological propertiesActa Biochimica et Biophysica Sinica 53:10–18.https://doi.org/10.1093/abbs/gmaa112
Article and author information
Author details
Funding
National Institutes of Health (DP2AT010376)
- Medha M Pathak
National Institutes of Health (R01NS109810)
- Medha M Pathak
National Institutes of Health (R01HL143297)
- Ardem Patapoutian
Howard Hughes Medical Institute (GT11549)
- Jesse R Holt
- Medha M Pathak
George Hewitt Foundation for Medical Research
- Wei-Zheng Zeng
National Science Foundation (DMS1763272)
- Jesse R Holt
- Wei-Zheng Zeng
Simons Foundation (594598)
- Jesse R Holt
- Wei-Zheng Zeng
Howard Hughes Medical Institute
- Ardem Patapoutian
National Institutes of Health (R01AR051219)
- Ardem Patapoutian
National Institutes of Health (5P30AR075047-03)
- Medha M Pathak
The funders had no role in study design, data collection and interpretation, or the decision to submit the work for publication.
Acknowledgements
We thank Dr. Jamison Nourse and Gabriella Bertaccini for technical support, Vivian Leung for help with illustrations, Dr. Ghaidaa Kashgari for helpful discussions, and members of the lab for comments on the manuscript. This work was supported by NIH grants DP2AT010376, R01NS109810 and a seed grant funded through 5P30AR075047-03 to MMP; NIH grants R01HL143297 and R01AR051219 to AP, a James H Gilliam Fellowship for Advanced Study (GT11549) from the Howard Hughes Medical Institute to MMP and JRH, a postdoctoral fellowship from the George Hewitt Foundation for Medical Research to W-ZZ, and a seed grant to JRH and W-ZZ from the UCI NSF-Simons Center for Multiscale Cell Fate Research (funded by NSF grant DMS1763272 and a Simons Foundation grant 594598). AP is an investigator of the Howard Hughes Medical Institute.
Ethics
All studies were approved by the Institutional Animal Care and Use Committee of University of California at Irvine (protocol number AUP-19-184) and The Scripps Research Institute (protocol number 08-0136-4), as appropriate, and performed in accordance with their guidelines.
Copyright
© 2021, Holt et al.
This article is distributed under the terms of the Creative Commons Attribution License, which permits unrestricted use and redistribution provided that the original author and source are credited.
Metrics
-
- 6,509
- views
-
- 1,025
- downloads
-
- 91
- citations
Views, downloads and citations are aggregated across all versions of this paper published by eLife.
Download links
Downloads (link to download the article as PDF)
Open citations (links to open the citations from this article in various online reference manager services)
Cite this article (links to download the citations from this article in formats compatible with various reference manager tools)
Further reading
-
- Cell Biology
- Developmental Biology
Eukaryotic cells depend on exocytosis to direct intracellularly synthesized material toward the extracellular space or the plasma membrane, so exocytosis constitutes a basic function for cellular homeostasis and communication between cells. The secretory pathway includes biogenesis of secretory granules (SGs), their maturation and fusion with the plasma membrane (exocytosis), resulting in release of SG content to the extracellular space. The larval salivary gland of Drosophila melanogaster is an excellent model for studying exocytosis. This gland synthesizes mucins that are packaged in SGs that sprout from the trans-Golgi network and then undergo a maturation process that involves homotypic fusion, condensation, and acidification. Finally, mature SGs are directed to the apical domain of the plasma membrane with which they fuse, releasing their content into the gland lumen. The exocyst is a hetero-octameric complex that participates in tethering of vesicles to the plasma membrane during constitutive exocytosis. By precise temperature-dependent gradual activation of the Gal4-UAS expression system, we have induced different levels of silencing of exocyst complex subunits, and identified three temporarily distinctive steps of the regulated exocytic pathway where the exocyst is critically required: SG biogenesis, SG maturation, and SG exocytosis. Our results shed light on previously unidentified functions of the exocyst along the exocytic pathway. We propose that the exocyst acts as a general tethering factor in various steps of this cellular process.