Clp protease and antisense RNA jointly regulate the global regulator CarD to mediate mycobacterial starvation response
Abstract
Under starvation conditions, bacteria tend to slow down their translation rate by reducing rRNA synthesis, but the way they accomplish that may vary in different bacteria. In Mycobacterium species, transcription of rRNA is activated by the RNA polymerase (RNAP) accessory transcription factor CarD, which interacts directly with RNAP to stabilize the RNAP-promoter open complex formed on rRNA genes. The functions of CarD have been extensively studied, but the mechanisms that control its expression remain obscure. Here, we report that the level of CarD was tightly regulated when mycobacterial cells switched from nutrient-rich to nutrient-deprived conditions. At the translational level, an antisense RNA of carD (AscarD) was induced in a SigF-dependent manner to bind with carD mRNA and inhibit CarD translation, while at the post-translational level, the residual intracellular CarD was quickly degraded by the Clp protease. AscarD thus worked synergistically with Clp protease to decrease the CarD level to help mycobacterial cells cope with the nutritional stress. Altogether, our work elucidates the regulation mode of CarD and delineates a new mechanism for the mycobacterial starvation response, which is important for the adaptation and persistence of mycobacterial pathogens in the host environment.
Editor's evaluation
CarD is an RNA polymerase interacting protein that is essential for mycobacterial viability, the levels of which are important for controlling gene expression in mycobacteria during various stress conditions. This study reports two mechanisms that regulate levels of CarD under stress conditions, including starvation. The authors report that CarD levels are tightly regulated and that there was a dramatic decrease in the levels of CarD when cells switched from the nutrient-rich to the starvation condition. They discovered two synergistic mechanisms that led to this dramatic decrease in CarD. The first is SigF-dependent induction of antisense RNA of CarD (AscarD), which inhibits CarD translation and a second mechanism involving Clp protease-mediated degradation of intracellular CarD. The work will be of interest to researchers studying non-coding RNAs, microbial gene expression, physiology and stress response.
https://doi.org/10.7554/eLife.73347.sa0Introduction
Bacterial starvation response refers to the physiological changes occurring in bacteria due to the lack of external nutrients during their growth and reproduction (Morita, 1982). Under starvation conditions, bacterial cells usually reduce the synthesis of rRNA and ribosome proteins (Gourse et al., 1996; Paul et al., 2004). The mechanisms of starvation response that have been elucidated in such bacteria as Escherichia coli and Bacillus subtilis work primarily by reducing rRNA transcription via decreasing the stability of the transcription initiation complex (Gourse et al., 2018; Hauryliuk et al., 2015).
Mycobacterium is a widespread genus of Gram-positive bacteria that comprises several important pathogens, including Mycobacterium tuberculosis, the causative agent of tuberculosis, which kills ~1.5 million people every year. One of the main difficulties in eliminating M. tuberculosis is that it usually responds to various host stresses, such as nutritional starvation, low oxygen, and low pH, by entering into a dormant state, which renders the organism extremely resistant to host defenses (Gengenbacher and Kaufmann, 2012). This genus also includes nonpathogens, such as M. smegmatis, which is widely used as a model organism for mycobacterial research. At present, the starvation response mechanisms of mycobacterial cells remain obscure.
Mycobacterial RNA polymerase (RNAP) is usually less efficient in forming RNAP-promoter open complex (RPo) than E. coli RNAP on the rRNA genes (Davis et al., 2015), and the RPo formed is rather unstable and readily reversible (Davis et al., 2015; Rammohan et al., 2015). To overcome this deficiency, mycobacterial cells have evolved two accessory transcription factors, CarD and RbpA, that help RNAP form a stable RPo (Hubin et al., 2017; Jensen et al., 2019; Rammohan et al., 2016). Both are global transcription factors that interact directly with RNAP to regulate the transcription of many downstream genes, including those of rRNA (Rammohan et al., 2016; Sudalaiyadum Perumal et al., 2018; Zhu et al., 2019). CarD stabilizes mycobacterial RPo via a two-tiered kinetic mechanism. First, CarD binds to the RNAP-promoter closed complex (RPc) to increase the rate of DNA opening; then, CarD associates with RPo with a high affinity to prevent the DNA bubble collapse (Davis et al., 2015; Hubin et al., 2017; Rammohan et al., 2015). Although binding of CarD to RNAP tends to increase the stability of RPo, it may also delay the dissociation of RNAP from the promoter region and thus hinder transcription progress (Jensen et al., 2019). Therefore, CarD may also inhibit the expression of certain genes. Whether CarD activates or inhibits the expression of a specific target gene appears to be determined by the kinetics of the initiation complex formation among CarD, RNAP, and the specific promoter (Jensen et al., 2019; Zhu et al., 2019). CarD was found to be essential for the survival of mycobacterial cells (Stallings et al., 2009) and weakening the interaction between CarD and RNAP rendered mycobacterial cells more sensitive to oxidative stress, DNA damage, and the effect of some antibiotics (Garner et al., 2014; Stallings et al., 2009; Weiss et al., 2012). A recent study showed that CarD regulates (either activates or inhibits) the expression of approximately two-thirds of genes in M. tuberculosis (Zhu et al., 2019). Despite the fact that CarD plays such a critical role in mycobacteria, the mechanisms that regulate its cellular levels remain largely uncharacterized.
It is worth noting that CarD was initially thought to inhibit the transcription of rRNA genes, and the transcription of carD was upregulated in response to starvation (Stallings et al., 2009). However, more recently, it was reported that CarD is a transcriptional activator of rRNA genes (Rammohan et al., 2015; Srivastava et al., 2013) and the growth rates of mycobacterial cells positively correlate with the CarD content (Garner et al., 2017; Stallings et al., 2009; Weiss et al., 2012). Nevertheless, the expression of CarD is still considered to be upregulated in response to starvation. If this was the case, the increased CarD would accelerate rRNA synthesis and mycobacterial growth under the starvation condition, which seems to contradict the current consensus (Irving and Corrigan, 2018; Rasouly et al., 2017; Srivatsan and Wang, 2008). Therefore, it is important to clarify the regulation of CarD expression under starvation conditions. In the current study, we found that although carD transcript levels were upregulated in response to starvation, its protein levels dramatically decreased. Further, we found that the reduction of CarD protein level under starvation conditions is a common regulatory mechanism that depends upon the functioning of both antisense RNA and Clp protease. This study describes the mechanisms behind the apparent contradiction between CarD mRNA and protein levels and reveals a new mechanism of mycobacterial response to stress.
Results
CarD protein level increases under genotoxic stresses but dramatically decreases under starvation conditions
CarD is an essential RNAP-interacting protein that regulates the transcription of rRNA genes and many related genes by stabilizing the RPo. While Stallings et al. found that the carD gene is upregulated in response to starvation and genotoxic stresses in M. smegmatis strain mc2155 (Stallings et al., 2009), they only monitored the transcriptional level but not the translation of carD, which may not truly reflect the CarD protein content. Therefore, to clarify the dynamics of CarD content under the starvation condition and genotoxic stresses, we examined both the carD transcript and CarD protein levels in the mc2155 strain by quantitative real-time PCR (qRT-PCR) and Western blot experiments, respectively. As shown in Figure 1, panels A and B, both carD transcript and CarD protein levels increased under genotoxic stresses triggered by H2O2, methyl methanesulfonate (MMS), or ciprofloxacin (CIP), which was consistent with the previous reports that CarD may be involved in DNA damage repair (Stallings et al., 2009). However, although the carD transcript level increased in response to starvation (Figure 1A), the CarD protein level, instead, decreased (marked by an arrow in Figure 1B). This observation indicates that the carD transcript level is upregulated in response to starvation, as previously reported (Stallings et al., 2009), but there are other mechanisms that down-regulate CarD protein level.
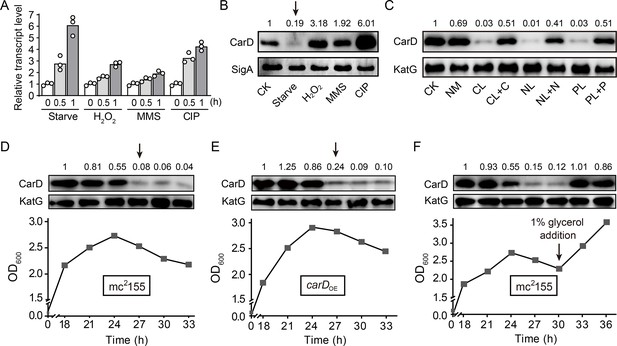
Changes of CarD transcript and protein levels under starvation and genotoxic stress.
(A, B) The transcript and protein levels of CarD, respectively, under different stress conditions. The carD transcript levels in the treated exponential mc2155 cells were measured by qRT-PCR, normalized to the sigA transcript levels, and expressed as the fold change of untreated cells. CK indicates the untreated cells of mc2155. ‘Starve’ means that mc2155 cells were first cultured in the 7H9 medium, and then transferred to phosphate-buffered saline (PBS) for 0.5 or 1 hr. For stimulation experiments, 10 mM H2O2, 0.1% methyl methanesulfonate (MMS), and 10 μg/ml of ciprofloxacin (CIP) were used. Individual data for the three biological replicates are shown in the corresponding columns. Western blot was used to detect the CarD protein levels under the same treatment conditions with SigA serving as the internal reference protein. (C) Protein levels of CarD under distinct starvation conditions. CK indicates the untreated exponential cells; NM indicates the exponential cells transferred into the normal medium for 4 hr; CL, NL, and PL indicate the exponential cells transferred into carbon-, nitrogen-, and phosphorus-limited media for 4 hr, respectively; CL + C, NL + N, and PL + P indicate the starved mc2155 cultures supplemented with the corresponding nutrients for 4 hr. KatG was used as the control in the Western blot experiments. CarD protein levels at the different growth stages in mc2155 (D, F), and carD overexpressing strain (carDOE, panel E). The lower part of the chart shows the respective growth curves with the sampling times. For panels (B–F), the number above each band of the Western blot represents their relative quantitative values, which are normalized with respect to their corresponding loading controls. For panels (B, D, and E), arrows above the Western blot results indicate the sharp decrease in CarD levels under starvation or stationary phase.
-
Figure 1—source data 1
Changes of carD transcript levels under starvation and genotoxic stress (numerical data for Figure 1A).
- https://cdn.elifesciences.org/articles/73347/elife-73347-fig1-data1-v2.xlsx
To investigate whether the decline in the CarD level is due to the lack of a specific nutrient or to a general response to starvation stress, we investigated the changes in CarD levels under carbon-, nitrogen-, and phosphorus-starvation conditions. We first cultured mc2155 cells to mid-exponential phase (MEP), harvested the cells, and then transferred these cells to the normal medium, carbon-limited, nitrogen-limited, and phosphorus-limited medium, followed by detecting the respective mRNA and protein levels of CarD. It is worth noting that although the carD transcript level increased in response to starvation conditions (Figure 1—figure supplement 1A), the CarD protein level decreased (Figure 1C). When the nutrient-limited media were supplemented with the corresponding nutrients, CarD returned to normal levels.
Since the mycobacterial cells in the stationary phase are in the state of nutritional starvation (Smeulders et al., 1999), we also measured the CarD protein levels at different growth periods of mc2155 cells. As shown in Figure 1D, the CarD level remained relatively constant in the exponential phase but dropped sharply in the early stationary phase (marked by an arrow in Figure 1D), which is consistent with the above starvation experiments. To further verify this result, we constructed a carD overexpressing strain (carDOE) (Figure 1—figure supplement 1B–D) and measured CarD protein levels at different growth periods. Interestingly, despite carD overexpression, the CarD protein level still decreased dramatically when the mycobacterial cells entered the stationary phase (marked by an arrow in Figure 1E). Since the carbon source in the culture medium was likely depleted when the mycobacterial cells entered the stationary phase (Smeulders et al., 1999), we speculated that the decrease in the CarD protein level could be caused by carbon starvation. To verify this hypothesis, we added 1% glycerol (glycerol is the main carbon source under normal culture conditions of M. smegmatis) to the mc2155 culture at the stationary phase and measured the CarD protein level 3 and 6 hr thereafter. As shown in Figure 1F, the CarD level significantly increased after the glycerol addition, and the mc2155 cells resumed normal growth. Considering that CarD activates the transcription of rRNA (Rammohan et al., 2015; Srivastava et al., 2013), and that cells need to reduce rRNA levels in response to starvation (Gourse et al., 2018), we believe that the reduction in the CarD level under starvation conditions may be an adaptive response of mycobacterial cells. Yet, when nutrients became available, CarD quickly returned to its normal level to allow the cells to resume growth.
CarD levels are dramatically decreased in M. bovis BCG and M. tuberculosis under host-like stress conditions
To investigate whether the significant reduction of CarD levels under starvation conditions also happens in other mycobacterial species, we carried out starvation experiments in two other mycobacteria, M. bovis BCG and M. tuberculosis H37Ra. The results are consistent with those in M. smegmatis, that is, CarD levels were all significantly reduced in response to carbon-, nitrogen-, and phosphorus-starvation conditions (Figure 2A, B). When nutrient-limited cultures were supplemented with the corresponding nutrients, CarD returned to the normal levels. In addition, we also measured the CarD levels at different growth phases of the two strains. As shown in Figure 2C, D, CarD levels were dramatically decreased when BCG and H37Ra cells entered the stationary phase, which is also consistent with the results in M. smegmatis. The above results indicate that the rapid reduction of the CarD level in response to starvation is a common phenomenon in mycobacteria, and regulating CarD content to cope with nutritional starvation is a conserved mechanism for the mycobacterial adaptive response.
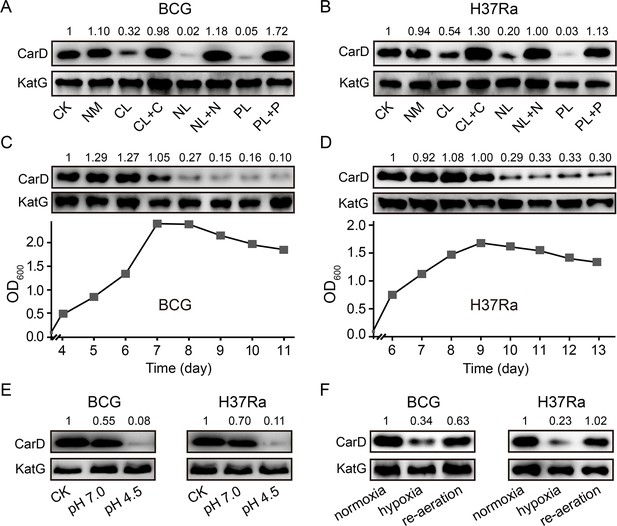
Changes of CarD levels in M.bovis BCG and M. tuberculosis H37Ra under host-like stress conditions.
(A, B) The protein levels of CarD in BCG and H37Ra strains, respectively, under distinct starvation conditions. (C, D) CarD protein levels at the different growth stages of BCG and H37Ra, respectively. (E) CarD protein levels in BCG and H37Ra under different pH conditions. (F) CarD protein levels in BCG and H37Ra under different oxygen availability conditions. For all panels, the number above each band of the Western blot represents their relative quantitative values, which are normalized with respect to their corresponding loading controls.
It is important to note that after infecting the host, pathogenic mycobacterial cells not only suffer from nutritional deprivation but are also exposed to hypoxic and acidic conditions. Therefore, to explore whether CarD plays a role in the adaptation of mycobacterial cells to the host environment, we measured CarD levels under these conditions. As shown in Figure 2E, CarD levels significantly decreased when the mycobacterial cells were transferred to the low pH media. For the hypoxic conditions, similarly, CarD levels were also reduced when mycobacterial cells were under hypoxic stress and returned to normal after the cultures were reaerated (Figure 2F and Figure 2—figure supplement 1). These results suggest that mycobacterial cells reduce CarD levels in response to host stresses to slow down their translation and metabolic rates, which likely contributes to the adaptation of pathogenic mycobacteria to the hostile environment.
Clp protease degrades CarD under starvation conditions
Since the CarD protein level decreased dramatically under nutritional starvation, hypoxic, and acidic conditions, we speculated that CarD might be proteolytically degraded. Clp is a special energy-dependent protease that regulates the response to various stresses (Michel et al., 2006; Raju et al., 2012; Schultz et al., 2017). The typical Clp proteolytic complex is formed by the association of ClpP, the main proteolytic unit, with an AAA+ (ATPases associated with a variety of cellular activities) unfoldase, either ClpX or ClpA/ClpC (Kirstein et al., 2009). Unlike most other bacteria, mycobacteria harbor two ClpP isoforms (ClpP1 and ClpP2), which associate with each other to form the ClpP1P2 heterotetradecamers (Akopian et al., 2012; Li et al., 2016). Through a quantitative proteomics approach, Raju et al. found that the CarD protein level in the clpP2 conditional deletion mutant was upregulated (Raju et al., 2014). However, that study only measured CarD in the exponential phase, not in the stationary phase. Therefore, it was unclear whether Clp protease mediates the efficient degradation of CarD in the stationary phase. To address this question, we constructed a clpP2 conditional mutant (clpP2CM) (Figure 3—figure supplement 1) through the CRISPR/Cpf1-mediated gene editing strategy (Yan et al., 2017), in which clpP2 could be expressed normally only upon addition of 50 ng/ml anhydrotetracycline (ATc), but could not do so when ATc was absent.
To explore the role of Clp protease in CarD degradation, we conducted ClpP2 depletion experiments. The cells of the clpP2CM mutant and control cells (Ms/pRH2502-clpP2) were first cultured in ATc-containing medium to the late exponential phase (OD600 ≈ 1.5), then harvested, washed, and reinoculated in the fresh medium with or without ATc. The results showed that CarD was effectively degraded when the control cells entered the stationary phase, regardless of the presence of ATc (Figure 3A, B). In the clpP2CM strain, CarD was also effectively degraded in the stationary phase when ATc was added to induce the clpP2 expression (Figure 3C) but persisted when clpP2 was not induced (Figure 3D). These results indicate that ClpP2 was essential for the efficient degradation of CarD in the stationary phase.
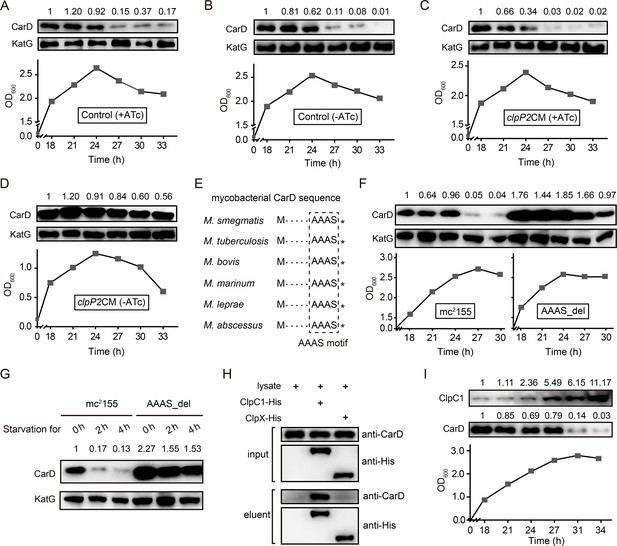
Clp protease is responsible for CarD degradation in the stationary phase.
(A–D) The cells were first cultured in anhydrotetracycline (ATc)-containing medium to the exponential phase (OD600 ≈ 1.5), then harvested, washed, and reinoculated in a fresh medium with or without ATc. 0 hr is the time when exponential cells were reinoculated into the fresh medium. (A, B) The intracellular CarD levels at different time points of the ATc-induced and ATc-uninduced control cells (Ms/pRH2502-clpP2), respectively. (C, D) The CarD levels at different time points of the ATc-induced and ATc-uninduced clpP2CM (clpP2 conditional mutant) cells, respectively. KatG was used as the control in the Western blot experiments. (E) Conservation of the LAAAS motif in mycobacterial CarD. The asterisk after the LAAAS motif indicates the stop codon. (F) CarD protein levels at the different growth stages of mc2155 and AAAS_del cells. (G) The starvation experiments of mc2155 and AAAS_del cells. (H) Verification of the interaction between CarD and ClpC1/ClpX by pull-down assay. (I) Protein levels of ClpC1 and CarD at different growth phases. For panels A–D, F–G, and I, the number above each band of the Western blot represents their relative quantitative values.
To investigate whether Clp protease is also required for degrading intracellular CarD under starvation conditions, we carried out a series of starvation experiments on clpP2CM cells harvested from the MEP. The results showed that CarD was effectively degraded when the ATc-induced clpP2CM cells were starved in PBS for 4 hr, while in the ATc-uninduced clpP2CM cells CarD was not degraded (Figure 3—figure supplement 2A, B). This result is consistent with the experimental data described above, allowing us to conclude that Clp protease was responsible for the degradation of CarD under starvation conditions.
Moreover, mycobacterial CarD contains a highly conserved C-terminal ‘LAAAS’ sequence (Figure 3E), which is similar to the Clp protease recognition motif (Gallego-García et al., 2017; Hoskins and Wickner, 2006; Lunge et al., 2020). To study whether this region mediates the degradation of CarD by Clp protease under stress conditions, we deleted the ‘AAAS’ coding sequence from the M. smegmatis carD gene and checked the CarD protein levels under stationary phase and starvation conditions. The results show that CarD in mc2155 is almost completely degraded under stress conditions, while CarD in the ‘AAAS’ deletion mutant (AAAS_del) is still highly retained (Figure 3F, G). This indicates that the deletion of the ‘AAAS’ motif largely prevented the Clp protease from degrading CarD. These results further strengthen the notion that Clp protease degrades CarD under starvation conditions.
Additionally, the efficient degradation of large proteins by Clp protease requires their unfolding in the presence of an AAA+ unfoldase (Akopian et al., 2012; Schmitz and Sauer, 2014). Mycobacteria harbor two functional Clp-associated unfoldases, ClpX and ClpC1 (Li et al., 2016; Schmitz and Sauer, 2014). Previous proteomics data showed that the CarD protein level is significantly upregulated when ClpC1 is depleted, suggesting that CarD is a substrate of ClpC1 (Lunge et al., 2020). To further confirm this result, we carried out an additional pull-down assay. The results showed that CarD does interact directly with ClpC1, but not with ClpX (Figure 3H). Therefore, we believe that ClpC1 specifically mediated the degradation of CarD. Furthermore, to clarify why CarD was more effectively degraded in the stationary phase, we monitored the protein levels of ClpP1, ClpP2, and ClpC1. The results showed that the protein levels of ClpP1 and ClpP2 were relatively constant throughout the growth phase (Figure 3—figure supplement 2C, D), while the level of ClpC1 protein was significantly upregulated during the stationary phase (Figure 3I). Since ClpC1 is the ATPase required for CarD recognition, unfolding, and translocation, its content likely determines the degradation efficiency of CarD. Taken together, these results suggest that the increase of ClpC1 level contributes to the efficient degradation of CarD during the stationary phase.
Starvation induces the transcription of antisense RNA of carD
Next, we wanted to know whether the intracellular CarD content is subject to other types of regulation other than degradation by Clp protease. After mining our previously published RNA-seq data of strain mc2155 (Li et al., 2017), we identified an antisense RNA transcribed from the antisense strand of the carD-ispD operon. As shown in Figure 4A, this antisense RNA (named AscarD) is partially complementary to the coding region of ispD but fully complementary to the coding region of carD. The RNA-seq data also showed that ascarD was specifically induced in the mid-stationary phase (MSP) (Figure 4A), and we confirmed this by RT-PCR (Figure 4—figure supplement 1A,B). Moreover, to determine the specific period when ascarD was induced, we examined the RNA level of AscarD throughout the growth phase, and the results showed that ascarD was induced at the onset of the stationary phase (Figure 4—figure supplement 1C).
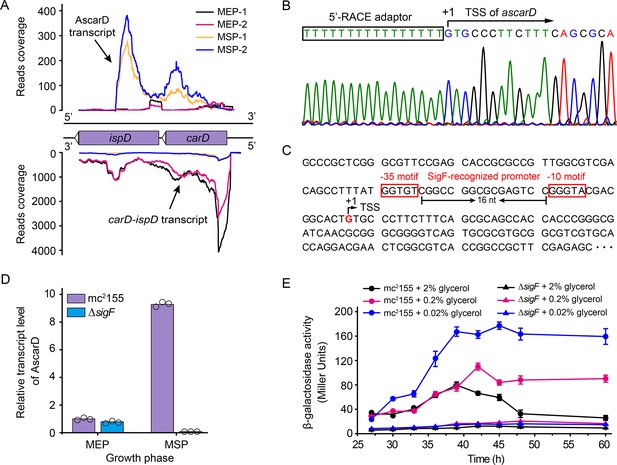
Identification and characterization of AscarD.
(A) Transcriptional landscapes of carD-ispD transcript and AscarD. Red and black lines represent exponential-phase cells, blue and green lines are from stationary-phase cells. Extensions of −1 and −2 represent two biological replicates. (B) Mapping of the transcriptional start site (TSS) of AscarD. The lower four-color chromatogram shows the results of Sanger sequencing, and the corresponding DNA sequence is displayed on the upper layer. The 5′-rapid amplification of cDNA ends (5′-RACE) adaptor sequence is framed by a black rectangle, and TSS is indicated by a black arrow. (C) Potential SigF-recognized −10 and −35 motifs upstream of the identified TSS are indicated with red rectangles. (D) AscarD transcript levels at different growth phases of mc2155 and ΔsigF strains were measured by qRT-PCR, normalized to sigA transcript levels, and expressed as fold change compared to levels of mc2155 cells at mid-exponential phase (MEP). Individual data for the three biological replicates are shown in the corresponding columns. (E) Promoter activities of ascarD in mc2155 and ΔsigF strains carrying a β-galactosidase-encoding reporter plasmid. Error bars indicate the standard deviation of three biological replicates.
-
Figure 4—source data 1
AscarD transcript levels at different growth phases of mc2155 and ΔsigF strains (numerical data for Figure 4D).
- https://cdn.elifesciences.org/articles/73347/elife-73347-fig4-data1-v2.xlsx
-
Figure 4—source data 2
Promoter activities of ascarD in mc2155 and ΔsigF strains (numerical data for Figure 4E).
- https://cdn.elifesciences.org/articles/73347/elife-73347-fig4-data2-v2.xlsx
To better characterize AscarD, we determined its transcriptional start site (TSS) by carrying out the 5′-RACE (5′-rapid amplification of cDNA ends) experiment (Figure 4B). The TSS identified by 5′-RACE was consistent with that revealed by the RNA-seq data. We also discovered potential SigF-recognized −10 and −35 motifs upstream of the identified TSS (Hartkoorn et al., 2012; Hümpel et al., 2010; Figure 4C). SigF is an alternative sigma factor that is active in the stationary phase, which is consistent with the transcriptional pattern of AscarD, suggesting that the transcription of ascarD is controlled by SigF. To verify this hypothesis, we examined the transcriptional level of ascarD in a sigF mutant (ΔsigF). As shown in Figure 4D, only a very low AscarD level could be detected in the ΔsigF strain in the MEP, and transition to the MSP could not induce it either. These data indicate that the expression of ascarD is regulated by SigF.
Further, since ascarD was highly expressed during the stationary phase, we speculated that transcription of ascarD could be also subject to carbon starvation. To verify this idea, we carried out lacZ reporter assays to examine the ascarD promoter activity under different carbon source (glycerol) concentrations. As shown in Figure 4E, the ascarD promoter activity gradually increased as the glycerol concentrations decreased. This indicates that ascarD could indeed be induced under carbon starvation conditions; however, in the ΔsigF strain, the expression of ascarD did not respond to the glycerol concentration (Figure 4E). This indicates that the response of ascarD to low carbon requires the presence of SigF, which is consistent with the above results. Thus, we confirm that the transcription of ascarD was highly induced in response to starvation in a SigF-dependent manner.
AscarD inhibits biosynthesis of CarD protein
Expression of AscarD was highly induced in response to starvation, while the CarD protein level was sharply reduced, suggesting that AscarD could be involved in regulating carD expression. To clarify this issue, we carried out lacZ reporter assays. The −213 to + 1090 region, containing the promoter, 5′-UTR, and CDS of carD and the promoter of ascarD on the antisense strand (abbreviated as PUCP), was translationally fused to lacZ to construct the PUCP plasmid (Figure 5A), in which the expression of the carD-lacZ chimeric transcript was expected to be regulated by the cis-encoded AscarD. However, in the PUCPmut plasmid, the −10 motif of ascarD is mutated (GGGTAC is mutated to GGGCGC) and could not transcribe AscarD, so the expression of the carD-lacZ transcript will not be affected by this antisense RNA. We then transformed the two plasmids into mc2155 cells and measured their β-galactosidase activities. As shown in Figure 5B, mycobacterial cells transformed with the PUCPmut plasmid exhibited higher β-galactosidase activity than those with the PUCP plasmid. This result indicates that AscarD repressed the expression of carD-lacZ transcript, and blocking the transcription of ascarD derepressed this regulation.
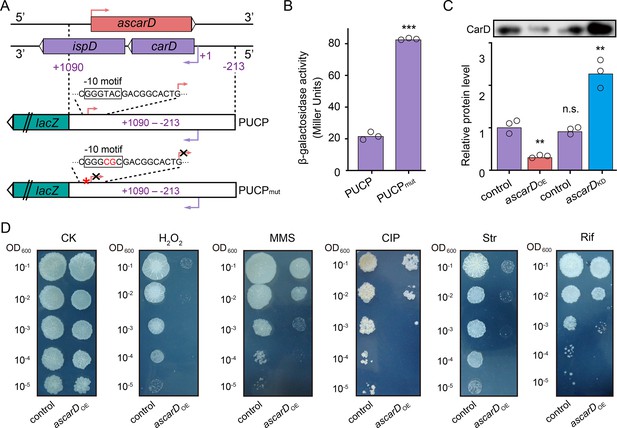
AscarD negatively regulates carD.
(A) A schematic diagram of PUCP and PUCPmut plasmids construction (see a detailed description in Experimental section). (B) β-Galactosidase activities of mc2155 strains transformed with PUCP or PUCPmut plasmid. Individual data for the three biological replicates are shown in the corresponding columns. (C) CarD protein levels in different strains. Mycobacterial cells were harvested at the mid-stationary phase (MSP). The upper part shows the Western blot with CarD levels, and the histogram below it shows the quantitative statistics of Western blot results. Statistical test was done using the Student's t-test, with ** indicating p-value <0.01, *** indicating p-value <0.001, and n.s. indicating p-value >0.05. (D) The tolerance of ascarDOE and control strains to oxidative stress, DNA damage, and antibiotic stimulation, respectively. Serially diluted bacterial suspensions were separately spotted onto normal 7H10 plate (CK) or plates containing 0.3 mM H2O2, 0.05% methanesulfonate (MMS), 0.2 μg/ml of ciprofloxacin (CIP), 0.1 μg/ml of streptomycin (Str), or 5 μg/ml of rifamycin (Rif), respectively.
-
Figure 5—source data 1
β-Galactosidase activities of mc2155 strains transformed with PUCP or PUCPmut plasmid (numerical data for Figure 5B).
- https://cdn.elifesciences.org/articles/73347/elife-73347-fig5-data1-v2.xlsx
-
Figure 5—source data 2
CarD protein levels in different strains (numerical data for Figure 5C).
- https://cdn.elifesciences.org/articles/73347/elife-73347-fig5-data2-v2.xlsx
To further explore the regulatory role of AscarD on carD expression, we overexpressed ascarD on a multiple-copy plasmid to construct ascarD high-expressing strain (ascarDOE) and knockdown the transcription of ascarD to construct the ascarD low-expressing strain (ascarDKD, Figure 5—figure supplement 1A, B) and examined the changes of CarD protein levels in these strains. As shown in Figure 5C, compared to the control strain, the CarD level in the ascarDOE strain was reduced, while in the ascarDKD strain it was significantly increased. This result indicates that AscarD inhibits the synthesis of CarD, which is consistent with the lacZ reporter assay data described above.
In addition, previous studies showed that CarD-impaired mycobacterial cells are more sensitive to oxidative stress, DNA damage, and the effect of some antibiotics (Garner et al., 2014; Stallings et al., 2009; Weiss et al., 2012). To further investigate the effect of AscarD on CarD expression and its biological function, we examined the tolerance of AscarD overexpression strain to the abovementioned stresses. The results showed that overexpression of AscarD significantly enhanced the sensitivity of mycobacterial cells to these stresses (Figure 5D). This result is consistent with the experimental data described above, allowing us to conclude that AscarD, when fully induced, significantly inhibits the expression of CarD and affects its function.
It is important to point out that the inhibitory effect of antisense RNA on target genes can occur at the post-transcriptional level (reducing transcript stability) and/or the translational level (inhibiting the transcript translation) (Georg and Hess, 2011). To determine the inhibition mode, we measured the transcript level of carD in the ascarDOE strain. The carD transcript levels were significantly higher in the ascarDOE strain than those in the control strain (Figure 5—figure supplement 1C), illustrating that overexpressed AscarD increases, rather than decreases, the stability of carD transcripts. Since AscarD only reduces the CarD protein level, but not the transcript level, we speculated that AscarD inhibits carD expression at the translational level.
Under starvation conditions, AscarD was highly induced to inhibit CarD protein synthesis. Since CarD protein levels are not only reduced during nutrient starvation, but also reduced under hypoxic and acidic conditions, we wanted to know whether ascarD is also induced under such stress conditions. To address this question, we monitored the RNA level of AscarD under the two stress conditions by qRT-PCR. The results showed that the AscarD level increased by 4.5 and 2.3 times in response to hypoxia and acid stress, respectively. These data indicate that AscarD was upregulated in response to a variety of stimuli to inhibit the protein synthesis of CarD and help mycobacterial cells adapt to the stress environment.
AscarD and Clp protease coregulate CarD-mediated mycobacterial adaptive response
AscarD and Clp protease regulate CarD at different levels. To explore which of these two regulations is dominant and whether there is a synergistic effect between the two, we examined the changes in CarD levels and bacterial survival rates in different mutant strains. As mentioned earlier, deletion of the ‘AAAS’ motif blocked the degradation of CarD by Clp protease. To block the regulation of CarD by AscarD, we mutated the promoter of ascarD in M. smegmatis genome and constructed a mutant strain, referred as AsM. In addition, to block the regulation of CarD by both AscarD and Clp protease, we also constructed a double mutant strain AsM/AAAS_del with a mutation in the promoter of ascarD and the deletion of the ‘AAAS’ motif of CarD. Next, we investigated the changes in CarD levels and bacterial survival of these strains under stress conditions. The Western blot results showed that, compared to the wild-type strain, CarD levels in the AsM strain slightly increased, while CarD levels in the AAAS_del strain increased significantly (Figure 6A). This indicates that under these stress conditions tested, Clp protease dominates the regulation of CarD levels.
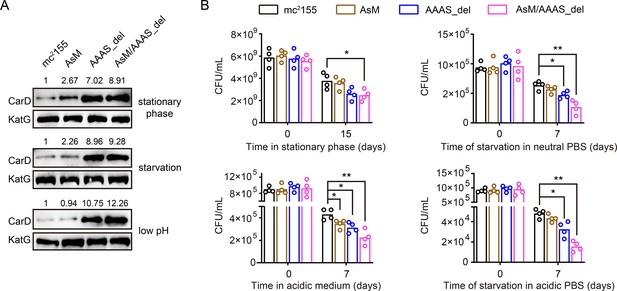
AscarD and Clp protease coregulate CarD-mediated mycobacterial adaptive response.
(A) Changes in CarD protein levels of different strains under various stress conditions. AsM, AAAS_del, and AsM/AAAS_del represent, respectively, AscarD promoter mutant, AAAS motif deletion, and double mutant strains. (B) Survival of different mycobacterial cells under various stress conditions. Statistical test was done using the Student’s t-test, with * indicating p value <0.05, and ** indicating p value <0.01.
-
Figure 6—source data 1
Survival of different mycobacterial cells under various stress conditions (numerical data for Figure 6B).
- https://cdn.elifesciences.org/articles/73347/elife-73347-fig6-data1-v2.xlsx
While bacterial survival assays showed that relieving the regulation of AscarD on CarD had a weak impact on the survival of mycobacterial cells, relieving the regulation of Clp protease on CarD had a moderate impact, and relieving both regulatory mechanisms strongly affected the survival of mycobacterial cells (Figure 6B). These results indicate that AscarD and Clp protease are both important for the survival of mycobacterial cells under stress conditions. While Clp protease is responsible for the rapid reduction of CarD protein levels, AscarD further reduces CarD protein levels by inhibiting carD translation. Their combined action helps mycobacterial cells save energy in the stress conditions by preventing the futile cycle of CarD synthesis and its degradation by Clp protease. Moreover, AscarD could prevent mycobacterial cells from overaccumulating CarD in the absence of the expression of Clp protease, which is essential for their survival under stress conditions. Altogether, AscarD and Clp protease work synergistically to decrease the CarD level to help mycobacterial cells respond to various stresses.
Discussion
In this paper, we present an in-depth study on the regulation of CarD expression and demonstrate that CarD is coregulated by AscarD antisense RNA and Clp protease under starvation conditions. Based on these results, along with those published by others, we propose a new mechanism for mycobacterial adaption to the starvation conditions, namely, that mycobacterial cells adjust their transcriptional and translational rates by regulating the CarD levels in response to the environmental conditions (Figure 7).
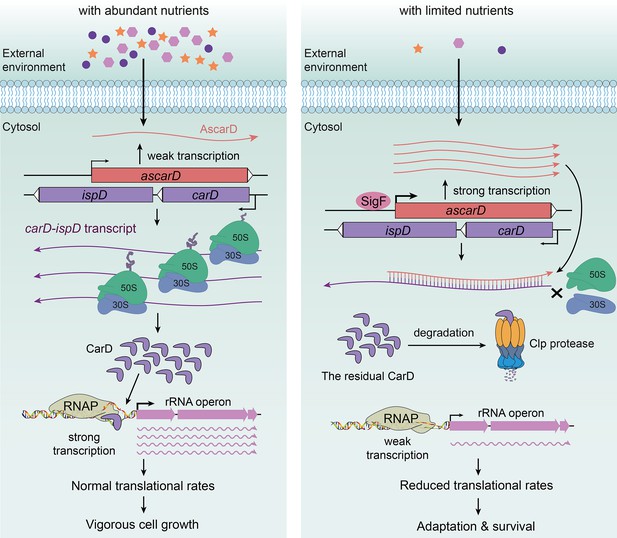
AscarD and Clp protease work together to regulate CarD-mediated starvation response.
The left and right panels represent the mycobacterial cells under nutrient-rich and nutrient-starved conditions, respectively.
Under abundant nutrition, mycobacterial cells use CarD to stabilize RPo (Bae et al., 2015; Davis et al., 2015; Rammohan et al., 2016; Rammohan et al., 2015), promoting the transcription of rRNA and other related genes (Garner et al., 2014; Rammohan et al., 2016; Srivastava et al., 2013) to ensure vigorous cell growth (Figure 7, left). However, when external nutrition gets scarce, SigF-regulated expression of AscarD is induced and it hybridizes with carD mRNA to prevent the translation of CarD protein (Figures 4 and 5). Meanwhile, the residual CarD protein is effectively degraded by the Clp complex to keep CarD at a very low level (Figures 1—3), which potentially reduces the stability of RPo and diminishes the synthesis of rRNA; these processes combine to slow down the rate of transcription and translation in mycobacterial cells (Figure 7, right). When nutrients are available, AscarD transcription is inhibited and carD mRNA gets translated to resume the normal CarD level and ensure the regrowth of mycobacterial cells (Figure 1F). Overall, these findings contribute to a better understanding of the mechanisms of mycobacterial adaptation to starvation and provide certain clues that might help in the treatment of tuberculosis.
Mycobacterial CarD defines a distinct adaptive response mechanism
Before this work, the best-known starvation response mechanism in Mycobacterium was the stringent response mediated by (p)ppGpp. Yet, its detailed mechanism is still not entirely clear today, although it is considered similar to the well-characterized stringent response mechanisms reported in E. coli and B. subtilis (China et al., 2012; Prusa et al., 2018; Weiss and Stallings, 2013). In E. coli, (p)ppGpp is synthesized in large quantities in response to starvation and directly interacts with RNAP to destabilize the RPo formed on rRNA genes and consequently reduces the rRNA synthesis (Gourse et al., 2018). However, (p)ppGpp in B. subtilis does not directly interact with RNAP but instead decreases the intracellular GTP content to destabilize the RPo formed on the genes that start from guanine, such as the rRNA genes (Kriel et al., 2012; Tojo et al., 2010). In mycobacteria, the exact effect and role of ppGpp on rRNA transcription are unclear, but (p)ppGpp likely inhibits the transcription of mycobacterial rRNA by affecting the stability of RPo (Prusa et al., 2018), which is similar to the CarD-mediated starvation response. Of course, these two mechanisms also have their unique features. First, (p)ppGpp reduces the stability of RPo (China et al., 2012; Tare et al., 2013), while CarD enhances its stability (Bae et al., 2015; Davis et al., 2015; Rammohan et al., 2016; Rammohan et al., 2015). Second, (p)ppGpp is rapidly synthesized in response to starvation, while CarD is effectively degraded under starvation conditions. Despite the differences between the two mechanisms, they basically work in the same way and ultimately help the mycobacterial cells adapt to starvation by reducing the rRNA synthesis. It should be noted that Stallings et al. previously reported that CarD is required for stringent response in M. smegmatis (Stallings et al., 2009). However, our data showed that CarD is effectively degraded under starvation conditions where stringent response usually occurs. This may seem to contradict the previous study, but since both CarD and (p)ppGpp interact with RNAP (Gourse et al., 2018; Stallings et al., 2009), the two molecules might have complex effects on RNAP that remain to be disentangled.
In addition, our study found that mycobacterial cells reduce CarD levels in response to hypoxic conditions. By analyzing the RNA-seq data for M. tuberculosis (Zhu et al., 2019), we found that 18 of the top 20 upregulated genes (Supplementary file 1) in CarDK125A strain (a mutant with predicted weakened affinity of CarD to DNA) belong to the previously identified dormancy regulon (Voskuil et al., 2003), and 31 out of 48 dormancy regulon genes are significantly upregulated. These data indicate that CarD represses the expression of dormancy regulon genes, and the reduction of CarD level during starvation or hypoxia may derepress these genes and facilitate mycobacterial dormancy. Since pathogenic mycobacteria usually live in a nutrient-deprived and hypoxic environment after infecting the host, we believe that CarD plays an important role in the dormancy and persistence of pathogenic mycobacteria in the host cells. Taken together, the CarD-mediated mycobacterial adaptive response mechanism is multifaceted; the reduction of CarD not only downregulates the transcription of rRNA to help mycobacterial cells adapt to nutritional starvation, but also enhances the expression of dormancy regulon genes to help pathogenic mycobacteria entering into a dormant state.
Efficient degradation of CarD during the stationary phase
The efficient degradation of CarD in the stationary phase may be caused by the increased expression of ClpC1. Notably, the increase in ClpC1 level during the stationary phase is also observed in Mycobacterium avium (Enany et al., 2021), and the content of ClpC or other AAA+ unfoldases (ClpA, ClpX, etc.) in many bacteria also increases significantly during the stationary phase (Chaussee et al., 2008; Cohen et al., 2006; Laakso et al., 2011; Michel et al., 2006; Sowell et al., 2008). This indicates that upregulation of Clp protease may be a conserved regulatory mechanism for bacteria to cope with starvation stress. Additionally, Schmitz and Sauer, 2014 previously suggested that binding of an AAA+ unfoldase strongly stimulates the peptidase activity of ClpP1P2 and stabilizes the conformation of the active complex. Therefore, the increased ClpC1 level during the stationary phase not only accelerates the unfolding of CarD, but also enhances the proteolytic activity of ClpP1P2, which ultimately mediates the effective degradation of CarD.
Considering the complexity of intracellular regulation, we speculate that there may be other reasons for the efficient degradation of CarD during the stationary phase. First, in the exponential phase, CarD may be protected by a certain protein complex. Garner et al. previously suggested that CarD–RNAP interaction protects CarD from proteolytic degradation (Garner et al., 2017). Therefore, RNAP (or other proteins) may protect CarD in the exponential phase. Then, after the mycobacterial cells enter the stationary phase, CarD would be detached from RNAP (or other proteins) through unknown mechanisms and be effectively degraded by Clp protease. Second, besides Clp protease, degradation of CarD may require an adaptor protein. For example, in Caulobacter crescentus, CpdR directly controls PdeA degradation by acting as a phosphorylation-dependent adaptor protein for the ClpXP protease (Abel et al., 2011). We speculate that there is possibly an adaptor protein that recognizes CarD under the starvation condition and delivers it to the Clp protease for degradation. Third, degradation of CarD by Clp protease may be affected by its modification. For example, certain protein substrates in B. subtilis are degraded by Clp protease only after their arginine residues are phosphorylated (Trentini et al., 2016). CarD might undergo a similar structural modification under the starvation condition, which is specifically recognized and degraded by Clp protease.
Role of AscarD in inhibition of the synthesis of CarD protein
The inhibitory effect of antisense RNAs on target genes generally occurs at the post-transcriptional level and/or the translational level (Georg and Hess, 2011). At the translation level, antisense RNAs mainly regulate the initiation of translation by blocking the SD sequence or adjacent regions of the target mRNA (Georg and Hess, 2018; Saberi et al., 2016; Sesto et al., 2013). In this study, we found that AscarD inhibited the synthesis of CarD protein but at the same time increased the stability of carD mRNA. Therefore, we speculated that the inhibition of CarD protein synthesis by AscarD is likely to occur at the translation level. So how does AscarD inhibit carD mRNA translation? Does its 3′-end cover the SD sequence of carD mRNA? It should be noted that we failed to identify the 3′-end of AscarD through 3′-RACE, but some of our results showed that AscarD does extend to the region that blocks the SD sequence of carD mRNA. We think this may be the main way that AscarD affects CarD protein synthesis. Of course, in addition to inhibiting the translation of carD mRNA, AscarD may also affect the synthesis of CarD protein in other ways. For example, transcription and translation in mycobacteria appear to be coupled (Johnson et al., 2020), such that the lead ribosome potentially contacts RNAP and forms a supramolecular complex. Therefore, a head-on RNAP on the antisense strand may become an obstacle to the RNAP on the sense strand and the trailing ribosomes, which may affect the synthesis of CarD protein.
Clp protease degrades CarD at the post-translational level, while AscarD inhibits CarD synthesis at the translational level. This two-tier mechanism allows mycobacterial cells to tightly control the CarD level. For example, when the content of Clp is insufficient or its function is lost, CarD may not be efficiently degraded; in that case, AscarD could prevent overaccumulation of CarD by inhibiting its synthesis. In fact, Clp protease is responsible for degrading unfolded/misfolded proteins that accumulate during stress conditions (LaBreck et al., 2017) and contributes to the clearance of truncated peptides from stalled ribosomes (Gottesman et al., 1998). The amount of these ‘competitive substrates’ increases under stress, for example, at high temperatures (Fujihara et al., 2002), which may result in the insufficient degradation of CarD by Clp protease. Furthermore, some natural compounds have been reported to inhibit the activity of Clp protease (Moreno-Cinos et al., 2019; Raju et al., 2012), suggesting that mycobacterial cells may face reduced or lost activity of Clp during in vitro growth or after infection of the host. In such situations, AscarD would be particularly important. Additionally, the presence of AscarD also helps mycobacterial cells save energy by preventing the futile cycle of CarD synthesis in the starvation condition and its degradation by Clp protease, which may be harmful to mycobacterial survival. Taken together, our data show that AscarD works together with Clp protease to maintain CarD at the minimal level to help mycobacterial cells cope with the nutritional stress.
Regulation of carD at the transcriptional level
Previous reports showed that the transcription of carD is regulated by SigB, but carD can still be effectively transcribed in the sigB knockout strain (Hurst-Hess et al., 2019). Since the −10 elements recognized by SigA and SigB are somewhat similar in mycobacteria, we speculate that SigA and SigB jointly regulate the carD expression, with SigA as the primary σ-factor responsible for the basal transcription of carD, and SigB as an alternative σ-factor responsible for the stimulated transcription of carD under stress conditions, which may also be the reason for the increasing carD expression after treatment with DNA-damaging agents (Figure 1A, B).
In addition, in Rhodobacter, CarD negatively regulates its own promoter, and the negative effect mainly depends on the extended −10 element (TGN) and the adjacent spacer of the promoter (Henry et al., 2021). At present, it is unclear whether mycobacterial CarD is autoregulated. After analyzing carD promoters from 91 different mycobacterial species, we found that mycobacterial carD also contains a conserved extended −10 element (Figure 7—figure supplement 1). Considering that only a few ‘TANNNT’ motifs in mycobacteria are preceded with extended −10 element (Cortes et al., 2013; Henry et al., 2020), we speculate that the highly conserved extended −10 element in the carD promoter may play an important role in the maintaining and regulating its basal activity. Moreover, a specific feature (T-rich) in the spacer immediately upstream of the extended −10 element contributes greatly to the autoregulation of Rhodobacter CarD. In Mycobacterium, there is no similar spacer, but there is a highly conserved dinucleotide ‘CG’ immediately upstream of the extended −10 element (Figure 7—figure supplement 1). Based on this limited information, it is difficult to determine whether mycobacterial CarD is autoregulated. In addition, it is worth mentioning that there are two conserved regions upstream of the carD core promoter regions (Figure 7—figure supplement 1). We speculate that these sequences may play a role in regulating the expression of carD, but to our knowledge, no potential transcription factor that can bind to these two sequences has been identified. Future studies to explore the function of these conserved elements will help to fully elucidate the regulatory mechanism of CarD.
Materials and methods
Bacterial strains and growth condition
Request a detailed protocolE. coli strains were cultivated in lysogeny broth medium at 37°C. M. smegmatis mc2155 wild-type strain (Yang et al., 2012) and its derivatives were grown at 37°C in Middlebrook 7H9 medium supplemented with 0.5% (vol/vol) glycerol and 0.05% (vol/vol) Tween 80, or on Middlebrook 7H10 agar supplemented with 0.5% (vol/vol) glycerol. M. bovis BCG and M. tuberculosis H37Ra strains (Yang et al., 2018) were grown at 37°C in 7H9 medium supplemented with 0.5% glycerol, 0.05% Tween 80% and 10% OADC (oleic acid, albumin, dextrose, and catalase), or on Middlebrook 7H11 agar supplemented with 0.5% glycerol and 10% OADC. When required, antibiotics were added at the following concentrations: kanamycin (Kan), 25 μg/ml; hygromycin (Hyg), 50 μg/ml; streptomycin (Str), 10 μg/ml. The strains used in this study are listed in Supplementary file 2.
Hartmans–de Bont (HDB) minimal medium, prepared according to reference (Smeulders et al., 1999), was used for starvation experiments. Briefly, 1 l of HDB medium contained: 10 mg of EDTA, 100 mg of MgCl2·6H2O, 1 mg of CaCl2·2H2O, 0.2 mg of NaMoO4·2H2O, 0.4 mg of CoCl2·6H2O, 1 mg of MnCl2·2H2O, 2 mg of ZnSO4·7H2O, 5 mg of FeSO4·7H2O, 0.2 mg of CuSO4·5H2O, 1.55 g of K2HPO4, 0.85 g of NaH2PO4, 2.0 g of (NH4)2SO4, 0.2% glycerol (vol/vol), and 0.05% Tween 80 (vol/vol). For the carbon starvation experiment, glycerol was removed; for the nitrogen starvation experiment, (NH4)2SO4 was removed; for the phosphorus-starvation experiment, both K2HPO4 and NaH2PO4 were removed, while 50 mM 3-(N-morpholino) propanesulfonic acid was added to replace lost buffering capacity.
Stimulation and starvation experiments
Request a detailed protocolMycobacterial cells were first grown to MEP in the normal 7H9 medium. For genotoxic reagent stimulation experiments, 10 mM H2O2, 0.1% MMS, and 10 μg/ml of CIP were separately added to the MEP mc2155 culture and maintained in roller bottle culture for additional 4 hr. For the PBS starvation experiment, the MEP mc2155 cells were harvested, resuspended in PBS supplemented with 0.05% Tween 80, and maintained in roller bottle culture for 4 hr. For the carbon-, nitrogen-, and phosphorus-starvation experiments, harvested MEP cells were resuspended in the HDB medium with carbon, nitrogen, or phosphorus removed, respectively, and maintained in roller bottle culture for 4 hr for the mc2155 cells, or 24 hr for the BCG and H37Ra cells. For the nutrient supplemented experiments, the abovementioned starved cultures were supplemented with the corresponding nutrients and maintained in roller bottle culture for additional 4 hr for the mc2155 cells, or 24 hr for the BCG and H37Ra cells. For the acid stimulation experiments, the harvested MEP cells were resuspended in the HDB medium with a low pH value (pH 4.5) and maintained in roller bottle culture for 4 hr for the mc2155 cells, or 24 hr for the BCG and H37Ra cells.
For anaerobic experiments, the modified Wayne model (Wayne and Hayes, 1996) was used. Briefly, 150 ml standard serum bottles containing 100 ml of 7H9 medium were used, in which methylene blue was added to the final concentration of 2 μg/ml to indicate oxygen content. The harvested MEP cells were reinoculated into the above serum bottles to make the final OD600 of 0.02. Then, the serum bottles were sealed with butyl rubber stoppers, closed tightly with screwcaps, and incubated at 37°C with shaking. The mc2155 cells were harvested 10 hr after the blue color disappeared completely, and the BCG and H37Ra cells were harvested 48 hr after the blue color completely disappeared. For the reaeration experiments, the abovementioned anaerobic cultures were transferred to roller bottles and harvested after the mycobacterial cells regrow.
RNA isolation, reverse transcription, and qRT-PCR
Request a detailed protocolThe total RNA was extracted by the TRIzol method using mycobacterial cells equivalent to 30 OD600 (e.g., 30 ml of a culture with OD600 of 1), as described previously (Li et al., 2017). The quality and concentration of total RNA were analyzed by NanoDrop 2000 (Thermo Scientific, USA). For reverse transcription, the total RNA was treated with DNase I (Takara Biotechnology, Japan) to remove any DNA contamination. The first-strand cDNA was synthesized using reverse transcriptase from the PrimeScript RT reagent kit (Takara Biotechnology, Japan) according to the manufacturer’s instructions. The cDNA of carD or ascarD was synthesized using gene-specific primers RT-carD-R or RT-ascarD-R instead of random primers, which allowed us to distinguish between the two transcripts. For qRT-PCR, the reaction was performed in ABI 7500 (Applied Biosystems, USA) under the following conditions: 95°C for 10 s, 60°C for 10 s, and 72°C for 10 s for 40 cycles. Relative quantification of gene expression was performed by the 2−ΔΔCT method (Livak and Schmittgen, 2001). sigA was used as a reference gene for the determination of relative expression. The primers used in this study are listed in Supplementary file 3.
Construction of the ascarD and carD overexpression strains
Request a detailed protocolThe overexpression plasmids of ascarD and carD were constructed based on the multicopy plasmid pMV261. For ascarD overexpression, we cloned the ascarD promoter and coding region into the pMV261 vector between the XbaI and HindIII restriction sites. For carD overexpression, we cloned the coding sequence into pMV261 between the EcoRI and EcoRV restriction sites, which allowed carD to be transcribed from the hsp60 promoter on the vector. The overexpression plasmids were then transformed into mc2155 cells to obtain the overexpression strains. The primers used are listed in Supplementary file 3.
CRISPRi-mediated gene knockdown strategy
Request a detailed protocolCRISPR/dCas9-mediated gene knockdown strategy (Singh et al., 2016) was carried out to construct the AscarDKD strain. Briefly, pRH2502 plasmid-containing int and dcas9 genes was integrated into the mc2155 genome to generate Ms/pRH2502 strain (Supplementary file 2). pRH2521 plasmid containing the small guide RNA (sgRNA) targeting ascarD was transformed into Ms/pRH2502 strain to obtain the final AscarDKD strain (Supplementary file 2). The expression of both dcas9 and sgRNA requires the induction by ATc. The transcription of the target gene (ascarD) was inhibited with the induction by 50 ng/ml of ATc, and the inhibition efficiency was assessed by qRT-PCR. It is worth noting that dCas9:sgRNA complex exhibits a strong inhibitory effect on the expression of a gene after it combines with the coding strand of the gene, but almost does not affect the expression of the gene when it combines with the template strand. The sgRNA we designed is combined with the coding strand of ascarD (i.e., the template strand of ispD), so it has a strong inhibitory effect on the transcription of ascarD (reduced 18.8 ± 2.6 times as quantitated by qRT-PCR) but has almost no effect on the transcription of ispD. The inhibition efficiency is shown in Figure 5—figure supplement 1A, B, and all related primers are listed in Supplementary file 3.
CRISPR/Cpf1-mediated mutagenesis
Request a detailed protocolCRISPR/Cpf1-mediated mutagenesis was carried out as described previously (Yan et al., 2017). For clpP2 conditional mutant (clpP2CM) construction, an exogenous clpP2 gene amplified with clpP2-F/R primer pair (Supplementary file 3) was ligated to the pRH2502 integration plasmid to obtain pRH2502-clpP2 recombinant plasmid, in which clpP2 is under the control of ATc-inducible promoter PUV15tetO. The pRH2502-clpP2 plasmid was then transformed and integrated into the mc2155 genome by attB–attP-mediated site-specific recombination, to obtain Ms/pRH2502-clpP2 strain. Finally, the endogenous clpP2 gene on Ms/pRH2502-clpP2 genome was mutated (pretranslational termination) using the CRISPR/Cpf1-mediated mutagenesis. Thus, clpP2 could be expressed normally in the clpP2CM strain only upon the addition of 50 ng/ml ATc, but could not do so when ATc was absent. It should be noted that, in principle, the clpP2CM strain cannot grow in the 7H9 medium without ATc. But when we first cultivated the clpP2CM cells to exponential phase in ATc-containing 7H9 medium, then harvested the cells, washed them, and inoculated them into ATc-free 7H9 medium, clpP2CM cells can grow slowly.
β-Galactosidase experiment
Request a detailed protocolFor PUCP construction, the −213 to +1090 region of carD, containing the carD promoter, 5′-UTR, carD CDS, and ascarD promoter on the antisense strand, was translationally fused to lacZ; for PUCPmut construction, the modified −213 to +1090 region of carD, containing the carD promoter, 5′-UTR, carD CDS, and the mutated ascarD promoter (GGGTAC was mutated to GGGCGC) on the antisense strand, was translationally fused to lacZ. Then the two plasmids were transformed into the mc2155 strain to measure the β-galactosidase activity. The detailed steps for β-galactosidase activity determination were carried out according to references (Ali et al., 2017; Tang et al., 2014).
5′-Rapid amplification of cDNA ends
Request a detailed protocolTo identify the TSS of ascarD, 5′-RACE analysis was performed with RNA extracted from mc2155 cells at midstationary phase grown in 7H9 medium. The 5′-RACE experiment was performed as described previously (Zaunbrecher et al., 2009). The primers used are listed in Supplementary file 3.
Western blot
Request a detailed protocolFor internal reference in the Western blot experiments, we used SigA or KatG as indicated. In the stress stimulation experiments (Figure 1B), SigA was used as an internal control because its level is not affected by the test stimuli. However, the SigA protein level in the stationary phase is significantly lower than that in the log phase (Gottesman et al., 1998), so when we studied the protein levels in several growth phases, SigA was not used as an internal control. After many tests, we found that the protein level of KatG remained basically unchanged throughout the growth stage, so KatG was used as an internal control in those experiments. (Note: KatG is highly induced under oxidative stress conditions, so it was not suitable for use as an internal control in the stress stimulation experiments.) CarD or KatG was detected using the CarD- or KatG-specific rabbit polyclonal antibodies prepared by Dia-An Biotech, Inc (Wuhan, China). For SigA detection, His × 6 tag was fused to the C-terminus of SigA by inserting its coding sequence immediately upstream of the sigA stop codon in the mc2155 genome, and the modified SigA-His × 6 protein was detected using rabbit polyclonal antibody to His × 6 (Yeasen Biotech Co., Shanghai, China). For Western blot assays, the amount of total protein loaded in each lane was the same, and the detailed procedures were as described previously (Hnasko and Hnasko, 2015). For quantification of Western blot results, Image J software was used. The intensities of bands in each lane were individually measured, and the intensities of the target protein were normalized with respect to their corresponding loading control. In each panel, the normalized value of the first sample was set to 1, and the values of other samples were represented by the fold changes of their normalized value relative to the first sample.
Pull-down assay
Request a detailed protocolThe His × 6 tagged ClpC1 (ClpC1-His) and ClpX (ClpX-His) recombinant proteins were expressed and purified from E. coli BL21(DE3). After purification, the eluate containing ClpC1-His/ClpX-His protein was dialyzed overnight at 4°C, then incubated with 1 mM ATP for 1 hr before loading onto the Ni-NTA resin. For pull-down assay, the resin-bound ClpC1-His or ClpX-His protein was separately incubated with lysate extracted from exponential mc2155 cells at room temperature for 30 min. The resins were washed with 50 mM imidazole for five times and eluted with 500 mM imidazole. The eluents were subjected to immunoblot assay using the antibodies indicated.
Bacterial survival assay
Request a detailed protocolFor the stationary-phase survival assay, mycobacterial cells were first grown to the stationary phase, followed by keeping them at 4°C, and the bacterial counts were performed on the 0th and 15th days thereafter. For stress survival assays, mycobacterial cells were first grown to the early-exponential phase (OD600≈ 0.5) and then diluted 50-fold into acidic 7H9 medium (pH = 4.5), neutral PBS (pH = 7.0), or acidic PBS (pH = 4.5). The dilutions were kept at 4°C, and the bacterial counts were performed 0th and 7th days thereafter. For genotoxic stress survival assay, ascarDOE and control cells were grown to MEP (OD600≈1.0), followed by diluting 101, 102, 103, 104, and 105 folds, respectively. Afterward, 3 μl of the bacterial suspension at each dilution level were separately spotted onto 7H10 plates containing either 0.3 mM H2O2, 0.05% MMS, 0.2 μg/ml of CIP, 0.15 μg/ml of Str, or 5 μg/ml of Rif, respectively. The plates were cultivated at 37°C for three days.
Statistical analysis
Request a detailed protocolStatistical testing was done using the Student’s t-test (two-tailed), with *** indicating p value <0.001, ** indicating p value <0.01, * indicating p value <0.05, and n.s. indicating p value >0.05. Error bars indicate standard deviation of three biological replicates. (Biological replicates represent tests performed on different biological samples representing an identical time or treatment dose, while technical replicates represent multiple tests on the same sample.)
Data availability
All data generated or analysed during this study are included in the manuscript and supporting file; Source Data files have been provided for Figures 1, 4, 5 and 6. These Source Data contain the numerical data used to generate the figures.
References
-
Growth phase-associated changes in the transcriptome and proteome of Streptococcus pyogenesArchives of Microbiology 189:27–41.https://doi.org/10.1007/s00203-007-0290-1
-
Mycobacterial RNA polymerase forms unstable open promoter complexes that are stabilized by CarDNucleic Acids Research 43:433–445.https://doi.org/10.1093/nar/gku1231
-
Mycobacterium tuberculosis: success through dormancyFEMS Microbiology Reviews 36:514–532.https://doi.org/10.1111/j.1574-6976.2012.00331.x
-
cis-antisense RNA, another level of gene regulation in bacteriaMicrobiology and Molecular Biology Reviews 75:286–300.https://doi.org/10.1128/MMBR.00032-10
-
Widespread Antisense Transcription in ProkaryotesMicrobiology Spectrum 6:RWR0029.https://doi.org/10.1128/microbiolspec.RWR-0029-2018
-
rRNA transcription and growth rate-dependent regulation of ribosome synthesis in Escherichia coliAnnual Review of Microbiology 50:645–677.https://doi.org/10.1146/annurev.micro.50.1.645
-
Transcriptional Responses to ppGpp and DksAAnnual Review of Microbiology 72:163–184.https://doi.org/10.1146/annurev-micro-090817-062444
-
Genome-wide definition of the SigF regulon in Mycobacterium tuberculosisJournal of Bacteriology 194:2001–2009.https://doi.org/10.1128/JB.06692-11
-
Recent functional insights into the role of (p)ppGpp in bacterial physiologyNature Reviews. Microbiology 13:298–309.https://doi.org/10.1038/nrmicro3448
-
Rhodobacter sphaeroides CarD Negatively Regulates Its Own PromoterJournal of Bacteriology 203:e0021021.https://doi.org/10.1128/JB.00210-21
-
The Western BlotMethods in Molecular Biology (Clifton, N.J.) 1318:87–96.https://doi.org/10.1007/978-1-4939-2742-5_9
-
Triggering the stringent response: signals responsible for activating (p)ppGpp synthesis in bacteriaMicrobiology (Reading, England) 164:268–276.https://doi.org/10.1099/mic.0.000621
-
Adapting the machine: adaptor proteins for Hsp100/Clp and AAA+ proteasesNature Reviews. Microbiology 7:589–599.https://doi.org/10.1038/nrmicro2185
-
Structure and Functional Properties of the Active Form of the Proteolytic Complex, ClpP1P2, from Mycobacterium tuberculosisThe Journal of Biological Chemistry 291:7465–7476.https://doi.org/10.1074/jbc.M115.700344
-
Transcriptome Landscape of Mycobacterium smegmatisFrontiers in Microbiology 8:2505.https://doi.org/10.3389/fmicb.2017.02505
-
Analysis of relative gene expression data using real-time quantitative PCR and the 2(-Delta Delta C(T)) MethodMethods (San Diego, Calif.) 25:402–408.https://doi.org/10.1006/meth.2001.1262
-
The unfoldase ClpC1 of Mycobacterium tuberculosis regulates the expression of a distinct subset of proteins having intrinsically disordered terminiThe Journal of Biological Chemistry 295:9455–9473.https://doi.org/10.1074/jbc.RA120.013456
-
ClpP Protease, a Promising Antimicrobial TargetInternational Journal of Molecular Sciences 20:E2232.https://doi.org/10.3390/ijms20092232
-
BookStarvation-survival of heterotrophs in the marine environmentIn: Morita RY, editors. Advances in Microbial Ecology. Springer. pp. 171–198.https://doi.org/10.1007/978-1-4615-8318-9
-
rRNA transcription in Escherichia coliAnnual Review of Genetics 38:749–770.https://doi.org/10.1146/annurev.genet.38.072902.091347
-
The stringent response and Mycobacterium tuberculosis pathogenesisPathogens and Disease 76:fty054.https://doi.org/10.1093/femspd/fty054
-
Bacterial proteolytic complexes as therapeutic targetsNature Reviews. Drug Discovery 11:777–789.https://doi.org/10.1038/nrd3846
-
CarD stabilizes mycobacterial open complexes via a two-tiered kinetic mechanismNucleic Acids Research 43:3272–3285.https://doi.org/10.1093/nar/gkv078
-
Cooperative stabilization of Mycobacterium tuberculosis rrnAP3 promoter open complexes by RbpA and CarDNucleic Acids Research 44:7304–7313.https://doi.org/10.1093/nar/gkw577
-
A Magic Spot in Genome MaintenanceTrends in Genetics 33:58–67.https://doi.org/10.1016/j.tig.2016.11.002
-
Natural antisense RNAs as mRNA regulatory elements in bacteria: a review on function and applicationsCellular & Molecular Biology Letters 21:6.https://doi.org/10.1186/s11658-016-0007-z
-
The excludon: a new concept in bacterial antisense RNA-mediated gene regulationNature Reviews. Microbiology 11:75–82.https://doi.org/10.1038/nrmicro2934
-
Adaptation of Mycobacterium smegmatis to stationary phaseJournal of Bacteriology 181:270–283.https://doi.org/10.1128/JB.181.1.270-283.1999
-
Proteomic analysis of stationary phase in the marine bacterium “Candidatus Pelagibacter ubique.”Applied and Environmental Microbiology 74:4091–4100.https://doi.org/10.1128/AEM.00599-08
-
Control of bacterial transcription, translation and replication by (p)ppGppCurrent Opinion in Microbiology 11:100–105.https://doi.org/10.1016/j.mib.2008.02.001
-
RbpA relaxes promoter selectivity of M. tuberculosis RNA polymeraseNucleic Acids Research 46:10106–10118.https://doi.org/10.1093/nar/gky714
-
Mycobacterium smegmatis BioQ defines a new regulatory network for biotin metabolismMolecular Microbiology 10:12817.https://doi.org/10.1111/mmi.12817
-
Inhibition of respiration by nitric oxide induces a Mycobacterium tuberculosis dormancy programThe Journal of Experimental Medicine 198:705–713.https://doi.org/10.1084/jem.20030205
-
Essential roles for Mycobacterium tuberculosis Rel beyond the production of (p)ppGppJournal of Bacteriology 195:5629–5638.https://doi.org/10.1128/JB.00759-13
-
CRISPR-Cas12a-Assisted Recombineering in BacteriaApplied and Environmental Microbiology 83:e00947-17.https://doi.org/10.1128/AEM.00947-17
-
A TetR-like regulator broadly affects the expressions of diverse genes in Mycobacterium smegmatisNucleic Acids Research 40:1009–1020.https://doi.org/10.1093/nar/gkr830
Article and author information
Author details
Funding
National Natural Science Foundation of China (31900057)
- Qing Tang
National Natural Science Foundation of China (32171424)
- Jin He
China Postdoctoral Science Foundation (2019M662654)
- Xinfeng Li
National Institutes of Health
- Michael Y Galperin
The funders had no role in study design, data collection, and interpretation, or the decision to submit the work for publication.
Copyright
This is an open-access article, free of all copyright, and may be freely reproduced, distributed, transmitted, modified, built upon, or otherwise used by anyone for any lawful purpose. The work is made available under the Creative Commons CC0 public domain dedication.
Metrics
-
- 1,800
- views
-
- 286
- downloads
-
- 14
- citations
Views, downloads and citations are aggregated across all versions of this paper published by eLife.
Download links
Downloads (link to download the article as PDF)
Open citations (links to open the citations from this article in various online reference manager services)
Cite this article (links to download the citations from this article in formats compatible with various reference manager tools)
Further reading
-
- Biochemistry and Chemical Biology
- Microbiology and Infectious Disease
Teichoic acids (TA) are linear phospho-saccharidic polymers and important constituents of the cell envelope of Gram-positive bacteria, either bound to the peptidoglycan as wall teichoic acids (WTA) or to the membrane as lipoteichoic acids (LTA). The composition of TA varies greatly but the presence of both WTA and LTA is highly conserved, hinting at an underlying fundamental function that is distinct from their specific roles in diverse organisms. We report the observation of a periplasmic space in Streptococcus pneumoniae by cryo-electron microscopy of vitreous sections. The thickness and appearance of this region change upon deletion of genes involved in the attachment of TA, supporting their role in the maintenance of a periplasmic space in Gram-positive bacteria as a possible universal function. Consequences of these mutations were further examined by super-resolved microscopy, following metabolic labeling and fluorophore coupling by click chemistry. This novel labeling method also enabled in-gel analysis of cell fractions. With this approach, we were able to titrate the actual amount of TA per cell and to determine the ratio of WTA to LTA. In addition, we followed the change of TA length during growth phases, and discovered that a mutant devoid of LTA accumulates the membrane-bound polymerized TA precursor.
-
- Microbiology and Infectious Disease
The persistence of latent viral reservoirs remains the major obstacle to eradicating human immunodeficiency virus (HIV). We herein found that ICP34.5 can act as an antagonistic factor for the reactivation of HIV latency by herpes simplex virus type I (HSV-1), and thus recombinant HSV-1 with ICP34.5 deletion could more effectively reactivate HIV latency than its wild-type counterpart. Mechanistically, HSV-ΔICP34.5 promoted the phosphorylation of HSF1 by decreasing the recruitment of protein phosphatase 1 (PP1α), thus effectively binding to the HIV LTR to reactivate the latent reservoirs. In addition, HSV-ΔICP34.5 enhanced the phosphorylation of IKKα/β through the degradation of IκBα, leading to p65 accumulation in the nucleus to elicit NF-κB pathway-dependent reactivation of HIV latency. Then, we constructed the recombinant HSV-ΔICP34.5 expressing simian immunodeficiency virus (SIV) env, gag, or the fusion antigen sPD1-SIVgag as a therapeutic vaccine, aiming to achieve a functional cure by simultaneously reactivating viral latency and eliciting antigen-specific immune responses. Results showed that these constructs effectively elicited SIV-specific immune responses, reactivated SIV latency, and delayed viral rebound after the interruption of antiretroviral therapy (ART) in chronically SIV-infected rhesus macaques. Collectively, these findings provide insights into the rational design of HSV-vectored therapeutic strategies for pursuing an HIV functional cure.