STAG2 promotes the myelination transcriptional program in oligodendrocytes
Abstract
Cohesin folds chromosomes via DNA loop extrusion. Cohesin-mediated chromosome loops regulate transcription by shaping long-range enhancer–promoter interactions, among other mechanisms. Mutations of cohesin subunits and regulators cause human developmental diseases termed cohesinopathy. Vertebrate cohesin consists of SMC1, SMC3, RAD21, and either STAG1 or STAG2. To probe the physiological functions of cohesin, we created conditional knockout (cKO) mice with Stag2 deleted in the nervous system. Stag2 cKO mice exhibit growth retardation, neurological defects, and premature death, in part due to insufficient myelination of nerve fibers. Stag2 cKO oligodendrocytes exhibit delayed maturation and downregulation of myelination-related genes. Stag2 loss reduces promoter-anchored loops at downregulated genes in oligodendrocytes. Thus, STAG2-cohesin generates promoter-anchored loops at myelination-promoting genes to facilitate their transcription. Our study implicates defective myelination as a contributing factor to cohesinopathy and establishes oligodendrocytes as a relevant cell type to explore the mechanisms by which cohesin regulates transcription.
Editor's evaluation
This manuscript will be of interest to scientists working on genome organisation and transcriptional control of myelination during mammalian brain development. The authors combine diverse and complementary experimental approaches to generate insights into how DNA looping contributes to transcriptional regulation in functionally specialised cell types. The experiments have been rigorously performed and the main conclusions are justified.
https://doi.org/10.7554/eLife.77848.sa0Introduction
Chromosomes in a single human diploid cell, if linearly stitched together, span a length of more than 2 m. They need to be properly folded to be housed in the cell nucleus with a diameter of 10 µm. Chromosome folding occurs in a dynamic, structured way that regulates gene expression, and DNA replication and repair. Initially discovered as the molecular glue that tethers sister chromatids for segregation during mitosis (Haarhuis et al., 2014; Uhlmann, 2016; Yatskevich et al., 2019; Zheng and Yu, 2015), the cohesin complex has later been shown to be critical for structured chromosome folding and gene expression (Haarhuis et al., 2017; Rao et al., 2017; Schwarzer et al., 2017; Wutz et al., 2017).
Cohesin is loaded on chromosomes by the cohesin loader NIPBL. The cohesin–NIPBL complex can extrude DNA loops bidirectionally in an ATP-dependent manner (Davidson et al., 2019; Kim et al., 2019; Vian et al., 2018). The chromatin insulator CTCF has been proposed to block loop extrusion by cohesin, establishing topologically associated domains (TADs) and marking TAD boundaries. Chromatin interactions within each TAD are favored whereas inter-TAD interactions are disfavored. Thus, chromosome loops and TADs shape long-range cis–element interactions, such as promoter–enhancer interactions, thereby regulating transcription.
The vertebrate cohesin complex contains four core subunits: the SMC1–SMC3 heterodimeric ATPase, the kleisin subunit RAD21 that links the ATPase heads, and the HEAT-repeat protein STAG1 or STAG2. STAG1 and STAG2 bind to RAD21 in a mutually exclusive manner and create docking sites for several regulatory proteins, including CTCF (Hara et al., 2014; Li et al., 2020). STAG1 and STAG2 also interact with DNA and the SMC1–SMC3 hinge domains (Shi et al., 2020). STAG1 and STAG2 play redundant roles in sister-chromatid cohesion in cultured human cells, as both need to be simultaneously depleted to produce overt cohesion defects (Hara et al., 2014).
Mutations of NIPBL and cohesin subunits, including STAG2, result in human developmental diseases termed cohesinopathies, which affect multiple organs and systems (Remeseiro et al., 2013b; Soardi et al., 2017). In patients with cohesinopathies, mental retardation and neurological abnormalities caused by brain development defects are common (Piché et al., 2019). Dysregulation of gene transcription as a result of reduced cohesin functions has been suggested to underlie these developmental defects (De Koninck and Losada, 2016; Remeseiro et al., 2013a). In addition, several cohesin genes, including STAG2, are frequently mutated in a variety of human cancers (Martincorena and Campbell, 2015).
In this study, we deleted Stag2 specifically in the nervous system in the mouse. The Stag2 cKO mice exhibited deficient myelination. Loss of STAG2 delayed the maturation of oligodendrocytes and reduced chromosome loops in oligodendrocytes and impaired the transcription of myelination-related genes. Our findings establish the requirement for cohesin in proper gene expression in specific cell types and implicate defective myelination as a potential contributing factor to cohesinopathy.
Results
Stag2 ablation in the nervous system causes growth retardation and neurological defects
Stag1 is required for mammalian embryonic development (Remeseiro et al., 2012), indicating that Stag2 cannot compensate for the loss of Stag1. To examine the physiological functions of Stag2 in the mouse, we created a Stag2 ‘floxed’ mouse line (Stag2f/f) by homologous recombination with a template that contained two LoxP sites flanking exon 8 (Figure 1A, B) and targeted a critical exon (exon 8) of Stag2, which is located on the X chromosome, using CRISPR–Cas9 (Figure 1—figure supplement 1A). The Stag2null embryos showed severe developmental defects and underwent necrosis by E11.5 days (Figure 1—figure supplement 1B). Thus, Stag2 is required for mouse embryonic development, consistent with a previous report (De Koninck et al., 2020). Stag1 and Stag2 have nonredundant developmental functions.
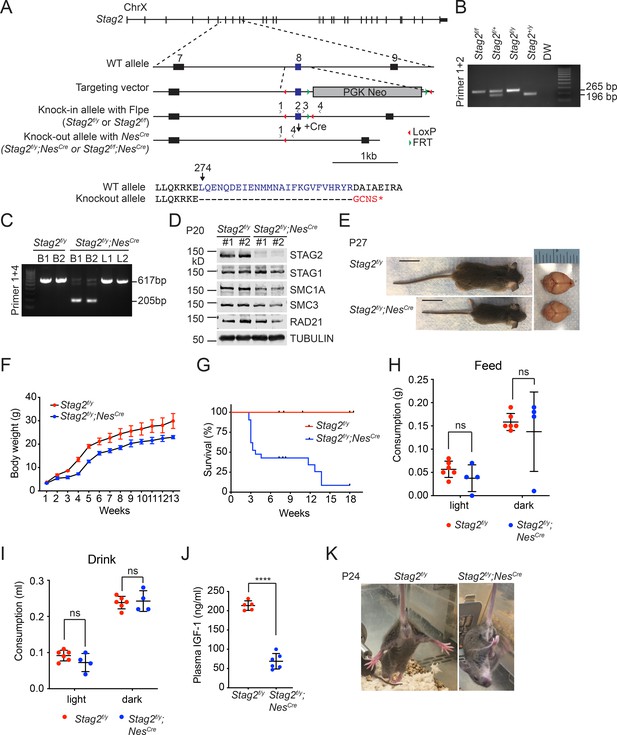
Stag2 ablation in the mouse nervous system causes growth retardation and neurological defects.
(A) Scheme for creating the ‘floxed’ Stag2 allele by gene targeting. The genomic structure of the wild-type (WT) Stag2 locus, the targeting vector, the knockin allele, the disrupted allele after Cre-mediated recombination, and the positions of the genotyping primers are shown. The amino acid sequence of the knockout allele in the targeted region is shown and aligned with that of the WT allele. (B) PCR analysis of the genomic DNA extracted from the tails of indicated mice with the primers in (A). (C) PCR analysis of genomic DNA extracted from brains (B) or livers (L) of indicated mice. (D) Immunoblots of brain lysates of Stag2f/y and Stag2f/y;NesCre mice with antibodies recognizing cohesin subunits and TUBULIN (as the loading control). (E) Representative images of Stag2f/y and Stag2f/y;NesCre mice. Scale bar = 2 cm. (F) Body weight of Stag2f/y and Stag2f/y;NesCre mice at different age. Mean ± standard deviation (SD) of at least three mice of the same age. (G) Survival curves of Stag2f/y (n = 12) and Stag2f/y;NesCre (n = 21) mice. Food (H) and water (I) consumption of 7- to 8-week-old Stag2f/y (n = 6) and Stag2f/y;NesCre (n = 4) mice. Mean ± SD; ns, not significant. (J) Plasma IGF-1 levels of 2-month-old Stag2f/y (n = 5) and Stag2f/y;NesCre (n = 6) mice. Mean ± SD; ****p < 0.0001. (K) Representative images of limb-clasping responses of Stag2f/y and Stag2f/y;NesCre mice. The uncropped images of blots in (B–D) are included in Figure 1—source data 1.
-
Figure 1—source data 1
Uncropped images of gels and blots in Figure 1.
- https://cdn.elifesciences.org/articles/77848/elife-77848-fig1-data1-v2.pdf
To study the functions of STAG2 in adult mice, we crossed the Stag2f/f mice with mice bearing the Rosa26CreErt2 genomic insertion and generated Stag2f/y;Rosa26CreErt2 progenies. The Stag2f/y;Rosa26CreErt2 adult mice were injected with tamoxifen to induce Stag2 deletion in the whole body (Figure 1—figure supplement 2A). Genotyping analysis of blood extracts showed that tamoxifen-induced efficient disruption of the Stag2 gene locus in Stag2f/y;Rosa26CreErt2 mice (Figure 1—figure supplement 2B, C). These Stag2-deficient adult mice did not show early onset of spontaneous tumor formation, indicating that Stag2 mutation alone in somatic cells of mice is insufficient to induce tumorigenesis. The Stag2-deficient mice also did not have other obvious adverse phenotypes (Figure 1—figure supplement 2D), except that they had slightly lower body weight (Figure 1—figure supplement 2E, F), probably due to tissue homeostasis alterations reported by others (De Koninck et al., 2020).
STAG2 mutations are found in human cohesinopathy patients with mental retardation and neuropsychiatric behaviors (Soardi et al., 2017). To study the function of STAG2 in the nervous system, we generated Stag2 conditional knockout mice (Stag2 cKO) by crossing Stag2f/f mice with Nestin-Cre mice (Giusti et al., 2014; Figure 1C, D). The progenies were born in the Mendelian ratio, but Stag2f/y;NesCre pups presented growth retardation and premature death (Figure 1E, G). More than 50% Stag2f/y;NesCre mice died aged about 3 weeks while the rest died at about 4 months. Stag2f/y mice did not show differences discernible from wild-type (WT) littermates. Although Stag2f/y;NesCre mice did not present microcephaly, they exhibited frequent hydrocephaly that might contribute to their premature death. The Stag2f/y;NesCre mice displayed normal drinking and feeding behaviors (Figure 1H), but showed reduced plasma IGF-1 levels compared to the control mice (Figure 1J). Stag2f/y;NesCre mice showed forepaw and hindlimb clasping (Figure 1K) and limb tremors (Video 1), which were not seen in Stag2f/y mice. These data indicate that Stag2 deficiency in the nervous system causes growth retardation and neurological defects.
Neurological defects of brain-specific Stag2 KO mice.
Stag2 ablation causes hypomyelination
Hematoxylin and eosin (H&E) staining of brain sections of Stag2f/y;NesCre mice did not reveal overt anatomical defects (Figure 2—figure supplement 1A). As revealed by immunohistochemistry assays using neuron- or astrocyte-specific antibodies, the differentiation of neurons and astrocytes in Stag2-deleted brains was largely normal (Figure 2—figure supplement 1B–E). To understand the origins of neurological defects caused by Stag2 deletion, we analyzed the gene expression changes in Stag2f/y;NesCre mouse brains at post-natal day 21 by RNA-sequencing (RNA-seq) (Figure 2A). Compared with the control groups, 105 and 62 genes were significantly down- or upregulated by more than twofolds, respectively, in the Stag2-deficient brains. The decreased expression of top differentially expressed genes (DEGs) was confirmed by reverse transcription quantitative PCR (RT-qPCR) (Figure 2B). Among the 105 downregulated DEGs in the brains of Stag2 cKO mice, 44 were enriched in myelin (Figure 2C; Thakurela et al., 2016). The ingenuity pathway analysis (IPA) pinpoints cholesterol biosynthesis pathways as the most affected canonical pathways (Figure 2D and Supplementary file 1). We further confirmed that the cholesterol biosynthesis precursors were reduced in Stag2f/y;NesCre brains (Figure 2—figure supplement 1F).
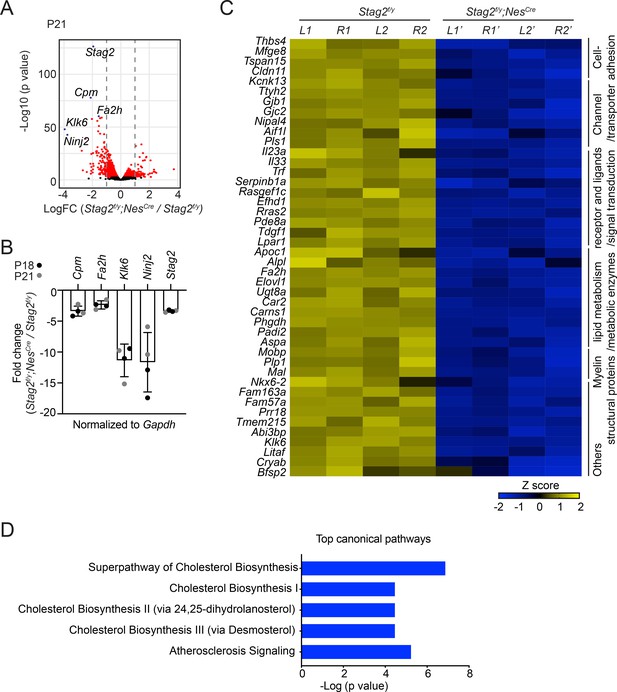
Stag2 ablation in mouse brains downregulates the expression of myelin genes.
(A) Volcano plot of bulk RNA-sequencing results of Stag2f/y and Stag2f/y;NesCre brain extracts. Top differentially expressed genes (DEGs) are colored blue and labeled. n = 4 pairs of P21 Stag2f/y and Stag2f/y;NesCre brain hemispheres were used for the comparison. (B) Reverse transcription quantitative PCR (RT-qPCR) analysis of the top downregulated genes in the brain extracts. n = 4 pairs of Stag2f/y and Stag2f/y;NesCre littermates were used. Mean ± standard deviation (SD). (C) Heatmap of the expression of myelin-enriched genes that were downregulated by more than twofolds in Stag2f/y;NesCre brains. L1 and R1, left and right brain hemispheres of the Stag2f/y#1 mouse. L2 and R2, left and right brain hemispheres of the Stag2f/y#2 mouse. L1’ and R1’, left and right brain hemispheres of the Stag2f/y;NesCre #1 mouse. L2’ and R2’, left and right brain hemispheres of the Stag2f/y;NesCre#2 mouse. The biological pathways of these genes are labeled on the right. (D) The top 5 canonical pathways identified by ingenuity pathway analysis (IPA) of the DEGs. The complete gene list is used as the background.
Myelin is the membrane sheath that wraps around axons to facilitate rapid nerve conduction and maintain metabolic supply (Williamson and Lyons, 2018). Dynamic myelination in the central nervous system (CNS) is critical for proper neurodevelopment, and defective myelination is associated with autoimmune and neurodegenerative diseases (Mathys et al., 2019; Wolf et al., 2021). Cholesterol biosynthesis is essential for normal myelination (Hubler et al., 2018; Saher et al., 2005). Ensheathment of neurons and gliogenesis were among the top enriched biological pathways in downregulated DEGs (Figure 2—figure supplement 2). The innate immune response was among the top enriched pathways in the upregulated DEGs. We hypothesized that depletion of STAG2 caused myelination defects in the nervous system.
Indeed, brain sections of Stag2f/y;NesCre mice showed greatly reduced luxol fast blue (LFB) staining compared to those of Stag2f/y and NesCre heterozygous mice at about P21 (Figure 3A and Figure 3—figure supplement 1A). Immunohistochemistry using antibodies against myelin proteins, Myelin basic protein (MBP) and Proteolipid protein 1 (PLP1), confirmed that Stag2 cKO mice had significant defects in myelin fiber formation at P18-P21 (Figure 3B–F). In both cerebral cortex and cerebellum, there were fewer and sparser myelin fibers in Stag2f/y;NesCre mice, as compared to the Stag2f/y controls. Axon myelin ensheathment was further examined using transmission electron microscopy (Figure 3G). Stag2f/y;NesCre mice at P18 had significantly fewer myelin-wrapped axons at optic nerves. Collectively, these data indicate insufficient myelination in the Stag2 cKO mice. Myelination predominantly occurs at 3 weeks after birth in the mouse. The timing of premature death of Stag2 cKO mice is thus consistent with defective myelination as a contributing factor to the lethality.
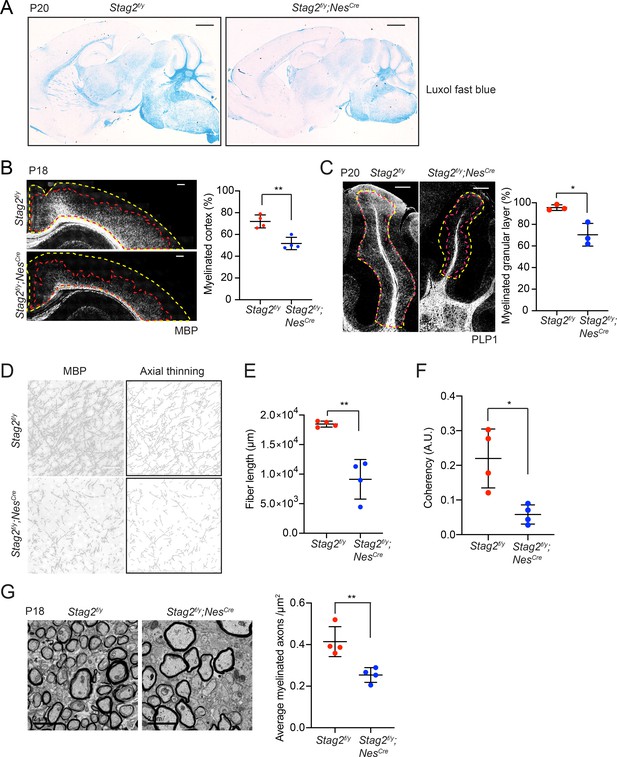
Stag2 ablation in the nervous system compromises myelination during early postnatal development.
(A) Luxol fast blue staining of the sagittal sections of Stag2f/y and Stag2f/y;NesCre brains. n = 3 animals per genotype. Scale bar = 1 mm. (B) Immunohistochemistry staining with the anti-MBP antibody in the cerebral cortex (left panel). Antibody-stained areas and DAPI staining regions are marked with red and yellow dashed lines, respectively. Scale bar = 200 μm. Quantification of the percentage of the myelinated cortex is shown in the right panel. n = 4 pairs of Stag2f/y and Stag2f/y;NesCre littermates were used (P18 or P21) for the comparison. **p < 0.01; mean ± standard deviation (SD). (C) Immunohistochemistry staining with the anti-PLP1 antibody in the cerebellum (left panel). Antibody-stained areas and DAPI staining regions are marked with red and yellow dashed lines, respectively. Scale bar = 200 μm. Quantification of the percentage of the myelinated cerebellum granular layer is shown in the right panel. n = 3 pairs of Stag2f/y and Stag2f/y;NesCre littermates were used (P20 or P25) for the comparison. *p < 0.05; mean ± SD. (D) Higher magnification images (left panel) of the immunohistochemistry staining with the anti-MBP antibody in (B). Images processed through axial thinning are shown in the right panel. Scale bar = 50 μm. Total fiber length (E) and fiber coherency (F) measured using the processed images in (D). n = 4 pairs of Stag2f/y and Stag2f/y;NesCre littermates were used (P18 or P21). *p < 0.05, **p < 0.01; mean ± SD. (G) Transmission electron microscopy images of the optic nerves (left panel). Scale bar = 2 μm. Quantification of myelinated axon distributions is shown in the right panel. n = 4 pairs of P18 Stag2f/y and Stag2f/y;NesCre littermates were used. n ≥ 10 fields of each mouse were taken, and the average distribution of myelinated axons were calculated for each mouse and plotted. **p < 0.01; mean ± SD.
We examined Stag1 and Stag2 expression patterns in P18 WT mouse brains by in situ hybridization using isotope-labeled RNA probes (Figure 3—figure supplement 1B). Both Stag1 and Stag2 were expressed at high levels in hippocampus, medial habenula, neocortex, and cerebellum granular layer. Aside from these regions, the Stag2 transcripts were detected at relatively high levels in subventricular zone, thalamus, fiber tracts, midbrain, and hindbrain regions. Stag2 is thus ubiquitously expressed in the brain.
STAG2 regulates transcription in OLs
Oligodendrocytes (OLs) are responsible for myelination in the CNS. To examine whether the OL lineage was affected by Stag2 deletion, we performed single-cell RNA-sequencing (scRNA-seq) analysis of Stag2f/y;NesCre and Stag2f/y forebrains at P13. As revealed by clusters in the t-SNE plot, the two genotype groups had similar cellular compositions (Figure 4A, B). All cell clusters were present in Stag2f/y;NesCre brains, again indicating generally normal neural cell differentiation. Cell-type identities were discovered with feature gene expression (Figure 4—figure supplement 1A). Based on the expression changes of Stag2 and other cohesin genes in OLs, astrocytes, and neuronal lineages (Figure 4—figure supplement 1B–D), it is clear that Stag2 ablation occurred early in the NPC stage and was maintained in all differentiated cell lineages.
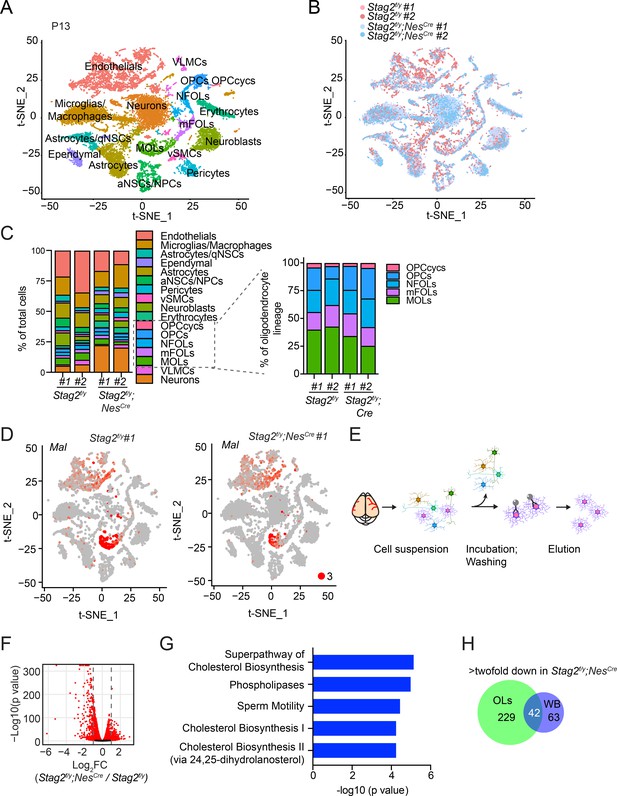
Deletion of Stag2 in mouse brains causes differentiation delay and transcriptional changes in oligodendrocytes.
(A) t-SNE plot of cell clusters in Stag2f/y and Stag2f/y;NesCre forebrains analyzed by single-cell RNA-sequencing (scRNA-seq). n = 2 mice of each genotype were used in the scRNA-seq analysis. aNSCs/NPCs, active neural stem cells or neural progenitor cells; Astrocytes/qNSCs, astrocytes or quiescent neural stem cells; OPCcycs, cycling oligodendrocyte (OL) progenitor cells; OPCs, OL progenitor cells; NFOLs, newly formed OLs; mFOLs, myelin-forming OLs; MOLs, matured OLs; VLMCs, vascular and leptomeningeal cells; vSMCs, vascular smooth muscle cells. (B) t-SNE clustering as in (A) but colored by genotype. (C) Left panel: cell-type composition and percentage as colored in (A). Right panel: percentage of cell clusters of the oligodendrocyte lineage. (D) FeaturePlot of a representative gene (Mal) specifically suppressed in MOLs of Stag2f/y;NesCre forebrains. A maximum cutoff of 3 was used. (E) Experimental scheme of the magnetic-activated cell sorting (MACS) of primary OLs. (F) Volcano plot of bulk RNA-sequencing (RNA-seq) results of Stag2f/y and Stag2f/y;NesCre primary OLs. (G) The top 5 canonical pathways identified by ingenuity pathway analysis (IPA) of the differentially expressed genes (DEGs) with more than twofold change in (F). The complete gene list is used as the background. (H) Commons DEGs shared between bulk RNA-seq analyses of the whole brains (WB) and primary OLs.
The OL lineage consisted of five clusters: cycling OL progenitors (OPCcycs), OL progenitors (OPCs), newly formed OLs (NFOLs), myelin-forming OLs (mFOLs), and fully matured OLs (MOLs). Quantification of the distributions of these five cell types within the OL lineage revealed a mild reduction in the proportion of MOLs in Stag2f/y forebrains (Figure 4C). We noticed that a higher percentage of neurons was recovered in the Stag2f/y;NesCre group. Since the bulk RNA-seq results did not show global upregulation of neuron-specific genes, we suspect that neurons in Stag2f/y;NesCre had fewer myelin-wrapped axons and were easier to be dissociated and kept alive during our library preparation for scRNA-seq. Thus, from the transcriptome analysis, we did not observe overt defects in most neural cell differentiation in the Stag2-deficient forebrain regions.
We then performed trajectory inference and pseudotime analysis of the OL lineage (Figure 4—figure supplement 2A, B). Consistent with our cell-type assignment, pseudotime variables indicated continuous differentiation from OPCs to NFOLs, mFOLs, and MOLs (Figure 4—figure supplement 2C,D). The reclustering of single cells in the OL lineage along the pseudotime path revealed that more cells were present in the terminal maturation stages in the Stag2f/y brains (Figure 4—figure supplement 2E, F). Conversely, more cells were retained at the undifferentiated stages in the Stag2f/y;NesCre brains. Strikingly, some myelination genes, including Myelin and lymphocyte protein (Mal), were specifically repressed in Stag2f/y;NesCre MOLs, with their expression in nonneural cells unaltered (Figure 4D and Figure 4—figure supplement 3A). These observations suggest that STAG2 deficiency delays the maturation of OLs and compromises myelination-specific gene expression in mature OLs. Interestingly, compared to Stag2 and genes encoding other cohesin core subunits, Stag1 transcripts are less abundant in the OL lineage, except for cycling OPCs (Figure 4—figure supplement 3B, C). The low expression of Stag1 in mature OLs might make these cells more dependent on Stag2 for function.
To confirm the transcriptional defects in the OL lineage caused by Stag2 deletion, we isolated primary OLs at intermediate differentiation stages from Stag2f/y;NesCre and Stag2f/y forebrains at P12-P14 with antibody-conjugated magnetic beads and conducted bulk RNA-seq analysis (Figure 4E). For both genotypes, the marker genes for NFOL and mFOLs were highly expressed in the isolated primary OLs (Figure 4—figure supplement 4A), suggesting that they mainly contained these two cell types. In Stag2-deleted OLs, 271 and 292 genes were downregulated or upregulated by more than two folds, respectively (Figure 4F and Supplementary file 2). Intriguingly, the downregulated genes were generally highly expressed in WT cells, whereas the upregulated genes had low expression levels in WT cells (Figure 4—figure supplement 4B–D). The top pathways enriched in the downregulated DEGs included the cholesterol and small molecule biosynthetic pathways and oligodendrocyte differentiation (Figure 4G and Figure 4—figure supplement 5). Cilium organization and assembly are the top enriched pathways in the upregulated DEGs (Figure 4—figure supplement 6). Among the 105 downregulated DEGs identified by RNA-seq analysis of the whole brain of Stag2-deficient mice, 42 were also differentially expressed in primary oligodendrocytes (Figure 4H). The cholesterol biosynthetic pathways were recognized as the major altered pathways (Figure 4—figure supplement 4E). Thus, defective cholesterol biosynthesis and oligodendrocyte differentiation likely underly hypomyelination and neurological defects in Stag2 cKO mice.
We performed chromatin immunoprecipitation sequencing (ChIP-seq) experiments to examine the enrichment of the active transcription mark H3K27ac in Stag2f/y and Stag2f/y;NesCre OLs and found that Stag2 loss did not appreciably affect H3K27Ac enrichment at transcription start sites (TSSs) (Figure 5A, B). Consistent with our RNA-seq results, the upregulated genes had much lower H3K27ac enrichment near their TSS, indicating that they were less active. We then checked the genomic distribution of STAG2 by ChIP-seq. Among other genomic loci, STAG2 was enriched at TSS of stable and downregulated genes, including genes in the cholesterol biosynthesis and myelination pathways (Figure 5C, D, Figure 5—figure supplement 1, and Supplementary file 2). Among the 271 downregulated DEGs, there were 210 genes (77%) with STAG2 enrichment near the transcriptional start site (TSS ± 2 kb). Thus, STAG2 occupied the promoter regions of many downregulated DEGs in oligodendrocytes. It was enriched at the TSS of upregulated genes to a lesser extent, with only 117 of the 292 (40%) upregulated DEGs exhibiting STAG2 ChIP-seq peaks at their TSS ± 2 kb regions. Stag2 loss might have indirectly affected the expression of these less active genes.
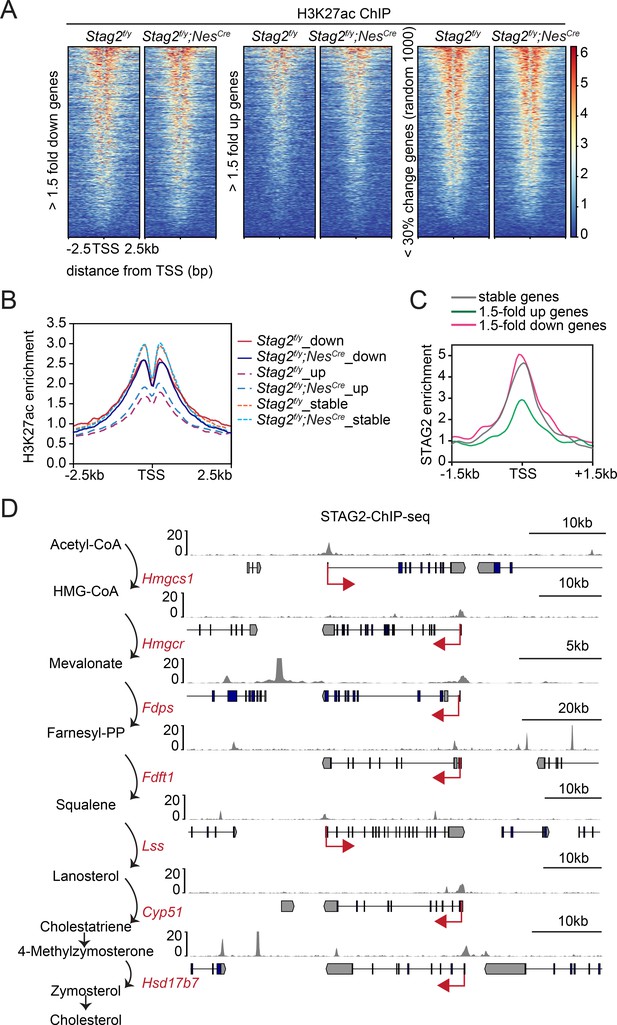
Enrichment of STAG2 and histone modifications at gene promoters.
(A) Heatmap of H3K27ac chromatin immunoprecipitation sequencing (ChIP-seq) signal enrichment in the promoter regions of genes in the indicated categories. (B) Density profile of H3K27ac ChIP-seq signal enrichment in the promoter regions of genes in the indicated categories as in (A). (C) Density profile of STAG2 ChIP-seq signal enrichment in the promoter regions of genes in the indicated categories as in (A). (D) Binding of STAG2 at the genomic loci of downregulated genes that encode cholesterol biosynthetic enzymes as revealed by ChIP-seq.
Stag2 deletion does not alter compartments or TADs in OLs
To investigate whether chromosome conformation was altered by Stag2 deletion and whether that caused transcription dysregulation, we performed high-dimensional chromosome conformational capture (Hi-C) analysis of primary OLs isolated from Stag2f/y and Stag2f/y;NesCre mice in biological replicates (Figure 6 and Figure 6—figure supplement 1). We observed few compartment switching events in Stag2-deleted cells (Figure 6A–C). Virtually all genomic regions in Stag2-deleted cells were kept in their original compartment categories (AA or BB) (Figure 6C). Only a very small number of genomic regions switched compartments (AB or BA). Consistent with the RNA-seq data, analysis of average gene expression changes of DEGs in these genomic regions revealed that more genes located in the transcriptionally active A compartment (AA) were repressed in Stag2-deleted cells and more genes in the transcriptionally silent B compartment (BB) were upregulated (Figure 6D and Figure 6—figure supplement 1C). Genes that switched from the A compartment to the B compartment were not more repressed compared to those that remained in the A compartment. Likewise, compared to genes that stayed in the B compartment, genes located in chromatin regions that switched from compartment B to A were not significantly activated. Acute depletion of all forms of cohesin eliminates TAD formation (Wutz et al., 2017). In contrast, deletion of Stag2 had minimal impact on TAD formation in oligodendrocytes (Figure 6E–G and Figure 6—figure supplement 1D), suggesting that STAG1-cohesin compensates for the loss of STAG2-cohesin in spatial organization of chromatin at larger than megabase scales. Therefore, our analyses did not uncover evidence for compartment switching and TAD alterations being the underlying cause for the observed gene expression changes in STAG2-deficient OLs.
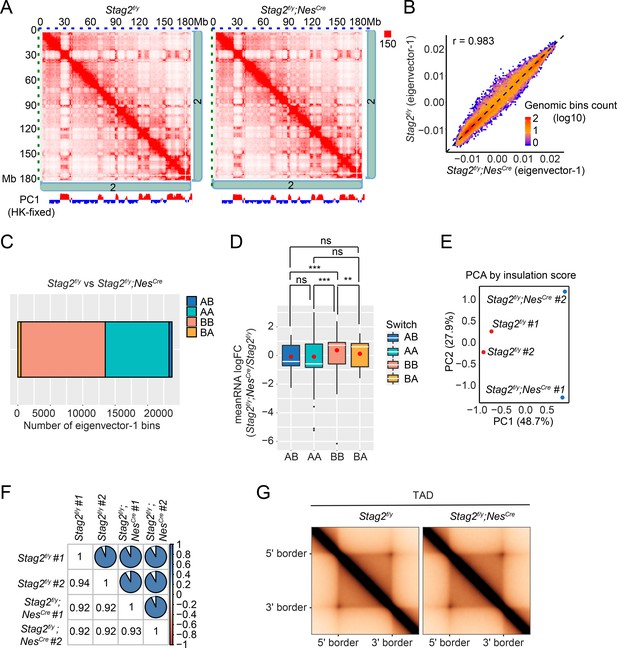
Loss of Stag2 does not alter compartments and topologically associated domains (TADs) in oligodendrocytes.
(A) Representative snapshots of balanced Hi-C contact matrices of chromosome 2. Tracks of eigenvector-1 fixed with housekeeping genes are shown below, with A and B compartments shown in red and blue, respectively. (B) Hexbin plot of eigenvector-1 for genomic bins (100 kb) in Stag2f/y and Stag2f/y;NesCre oligodendrocytes (OLs). (C) Chromatin bins were classified into four categories based on the eigenvector sign and whether it has switched with a delta bigger than 1.5. AB, changing from compartment A in Stag2f/y to compartment B in Stag2f/y;NesCre; BA, from B in Stag2f/y to A in Stag2f/y;NesCre; AA, A in both Stag2f/y and Stag2f/y;NesCre; BB, B in both Stag2f/y and Stag2f/y;NesCre. (D) Boxplot of averaged gene expression change of differentially expressed genes (DEGs) (RNA logFC cutoff of ±0.58) inside each genomic bin. Bins counted: AA, 1646; AB, 56; BA, 69; BB, 910. Red dots represent the mean value. An unpaired Wilcoxon test was used for the statistical analysis. **p < 0.01; ***p < 0.001; ns, not significant. Principal component (E) and similarity (F) analysis performed using the insulation score at 10 kb resolution. (G) Aggregate TAD analysis on the 10 kb merged Hi-C matrices using TADs called from the merged samples of Stag2f/y at 10 kb resolution.
Promoter-anchored loops were reduced in Stag2-deleted OLs
While TAD boundaries are largely conserved among species and cell types, chromatin interactions within each TAD are more flexible and variable in cells undergoing differentiation, tumorigenesis, and reprogramming (Dixon et al., 2015; Dixon et al., 2012). Among the intra-TAD chromatin interactions, the enhancer–promoter loops are particularly important for transcription and are often cell-type specific. We examined whether chromatin loops in OLs were affected by Stag2 loss. Compared to Stag2f/y OLs, Stag2f/y;NesCre OLs had significantly fewer loops across almost all genomic distances (Figure 7A, B and Figure 7—figure supplement 1). The common and genotype-specific loops are reproducible in each replicate. Loops specific to Stag2f/y;NesCre OLs, which were likely mediated by STAG1-cohesin, were longer than STAG2-dependent Stag2f/y-specific loops. When genomic distances exceeded 0.25 Mb, the loops from Stag2f/y;NesCre cells gradually gained higher scores over loops from Stag2f/y cells (Figure 7C). Therefore, STAG1-cohesin cannot completely compensate for STAG2-cohesin during loop formation. STAG1-cohesin-mediated loops are relatively longer than STAG2-cohesin-mediated loops, consistent with published findings in HeLa cells (Wutz et al., 2020).
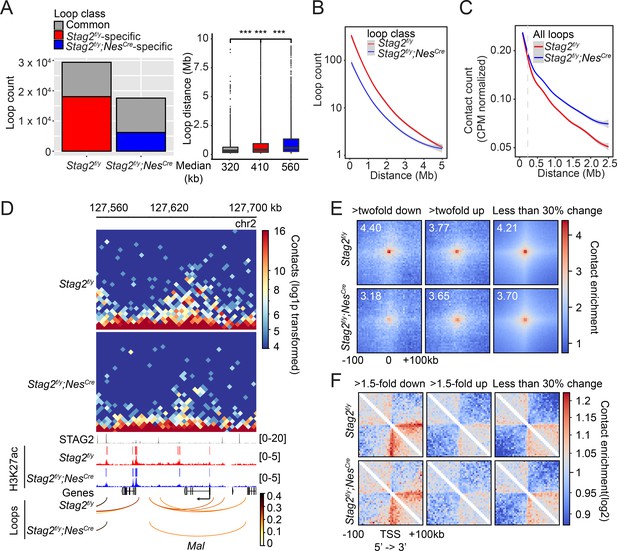
Stag2 deletion impairs the formation of total and promoter-anchored loops in oligodendrocytes.
(A) Loop counts (left panel) and length (right panel) in the indicated categories of Stag2f/y and Stag2f/y;NesCre oligodendrocytes (OLs). ***p < 0.001. (B) Loop counts plotted against loop length (from 0 to 5 Mb) of Stag2f/y and Stag2f/y;NesCre OLs. (C) Normalized contact counts for loops across different genomic distances in Stag2f/y and Stag2f/y;NesCre OLs. (D) Representative snapshots of contact maps at the Mal gene locus.hic files generated by HiC-Pro were converted to.cool format for plotting at 5 kb resolution. Tracks and narrow peaks from STAG2 and H3K27ac chromatin immunoprecipitation sequencing (ChIP-seq) as well as the loops are plotted below. Transcription direction is indicated by the black arrow. (E) Pile-up analysis of loop ‘dots’-centered local maps for the promoter-anchored loops of genes in the indicated categories. The maps are balanced, normalized by distance, and plotted at 5 kb resolution. The numbers indicate the enrichment of the central pixel over the upper left and bottom right corners. (F) Pile-up analysis of the local contact maps centered around the transcription start site (TSS) of genes in the indicated categories. Transcription directions are indicated below. 1000 stable genes are chosen randomly and used for the analysis. The maps are balanced, normalized by distance, and plotted at 5 kb resolution. Diagonal pixels are omitted.
We then tested whether the loop number decrease in Stag2-deficient cells could be a cause for transcriptional changes. When examining the local Hi-C maps, we noticed that loops anchored at gene promoters, including those of downregulated genes, were reduced in Stag2f/y;NesCre oligodendrocytes (Figure 7D, Figure 7—figure supplement 2A–C, and Supplementary file 2). The effects were again reproducible in each replicate (Figure 7—figure supplement 2D). Promoter-anchored loops (P-loops) can potentially be promoter–promoter links, promoter–enhancer links, and gene loops. The total number of P-loops was proportionally decreased in Stag2f/y;NesCre cells (Figure 7—figure supplement 3A). Moreover, the loops anchored at the downregulated genes were stronger than those at upregulated and stable genes (Figure 7—figure supplement 3B). We then compared P-loops associated with DEGs using pile-up analysis of local contact maps. Loop enrichment at promoters of downregulated genes was reduced in Stag2f/y;NesCre cells to a greater extent than that at promoters of upregulated and stable genes (Figure 7E). Among the 162 downregulated DEGs with reduced promoter-anchored loops in the Stag2-depleted cells, 137 genes (85%) had STAG2 peaks in their promoter regions (TSS ± 2 kb). The loops anchored at downregulated genes with STAG2 binding had significantly higher loop scores, compared to those with no STAG2 binding (Figure 7—figure supplement 3C, D). This difference was still observed in Stag2-deleted cells, suggesting that the stronger looping at these gene promoters might be maintained by STAG1-cohesin or other factors in these cells. By contrast, the loops anchored at upregulated genes with STAG2 binding had lower loop scores. These differences became insignificant in the Stag2-deleted cells. The loop scores of loops anchored at stable genes were not affected by STAG2 occupancy. Taken together, our results suggest that Stag2 loss diminishes the number of, but not the strength, short chromosome loops, including promoter-anchored loops. Highly expressed genes might be more reliant on these loops for transcription and are preferentially downregulated by Stag2 loss (Figure 4—figure supplement 4B-D).
We also performed pile-up analysis of local chromatin regions flanking TSSs (Figure 7F). Strikingly, we observed a clear stripe that extended from the TSS of downregulated gene only in the direction of transcription. The formation of promoter-anchored stripes (P-stripes) on aggregated plots is consistent with one-sided loop extrusion from the promoter to the gene body. The P-stripe was still present in Stag2f/y;NesCre cells, suggesting that STAG1 could compensate for the loss of STAG2 and mediate its formation (Figure 7F and Figure 7—figure supplement 3E).
Discussion
Cohesin is critical for the three-dimensional (3D) organization of the genome by extruding chromosome loops. Acute depletion of cohesin abolishes chromosome loops and TADs, but has moderate effects on transcription. The two forms of cohesin in vertebrate somatic cells, namely STAG1-cohesin and STAG2-cohesin, have largely redundant functions in supporting sister-chromatid cohesion and cell viability, but they have nonredundant functions during development. In this study, we have established a myelination-promoting function of STAG2 in the CNS in the mouse. We further provide evidence linking hypomyelination caused by STAG2 loss to reduced promoter-anchored loops at myelination genes in oligodendrocytes.
Myelination functions of STAG2 and implications for cohesinopathy
Selective ablation of Stag2 in the nervous system in the mouse causes growth retardation, neurological defects, and premature death. STAG2 loss delays the maturation of oligodendrocytes and reduces the expression of highly active myelin and cholesterol biosynthesis genes in oligodendrocytes, resulting in hypomyelination in the CNS. Hypomyelination disorders in humans and mice are known to produce abnormal neurological behaviors similar to those seen in our Stag2 cKO mice, suggesting that hypomyelination is a major underlying cause for the phenotypes in Stag2 cKO mice. The growth retardation in these mice can be explained by insufficient secretion of growth hormones, which may be a consequence of defective neuronal signaling.
Mutations of cohesin subunits and regulators, including STAG2, cause the Cornelia de Lange syndrome (CdLS) and other similar developmental diseases, collectively termed cohesinopathy. CdLS patients exhibit short stature and developmental defects in multiple tissues and organs, including the brain. Although STAG2 mutations are implicated in human cohesinopathy, these mutations are rare and hypomorphic (Soardi et al., 2017). The cohesin loader NIPBL is the most frequently mutated cohesin regulator in cohesinopathy (Mannini et al., 2013). NIPBL deficiency is expected to affect the functions of both STAG1- and STAG2-cohesin. It is possible that the partial loss of STAG2-cohesin function leads to subtle myelination defects in patients with cohesinopathy. Indeed, lack of myelination in certain brain regions of CdLS patients has been reported (Avagliano et al., 2017; Vuilleumier et al., 2002). As myelination of the CNS mostly occurs after birth and during childhood, strategies aimed at enhancing myelination might help to alleviate certain disease phenotypes and symptoms.
Mechanisms by which STAG2 promotes myelination
STAG2 promotes oligodendrocyte maturation and the expression of myelination genes in mature oligodendrocytes. Because STAG2 does not have an established cohesin-independent function, it most likely activates the myelination-promoting transcriptional program as a core component of cohesin. Consistent with previous reports (Rao et al., 2017), loss of STAG2-cohesin in oligodendrocytes does not affect genome compartmentalization, but reduces the number of relatively short chromosome loops, including promoter-anchored loops. Promoter-anchored loops at downregulated genes are reduced to a greater extent than those at stable and upregulated genes. These findings suggest that STAG2-cohesin promotes the myelination transcriptional program by forming promoter-anchored loops.
Pile-up analysis of Hi-C maps reveals the formation of asymmetric promoter-anchored stripes in the direction of transcription at downregulated genes, indicative of active loading of cohesin at TSSs followed by one-sided loop extrusion from the promoter to the gene body. The stripes are, however, not reduced in STAG2-deficient cells. Because both forms of cohesin are capable of loop extrusion, it is possible that STAG1-cohesin can compensate for the loss of STAG2-cohesin in loop extrusion. It remains to be tested whether the intrinsic kinetics and processivity of loop extrusion mediated by the two forms of cohesin are differentially regulated by cellular factors or posttranslational modifications and whether these differences contribute to their nonredundant roles in transcription regulation.
We envision three possibilities that may account for why oligodendrocytes, but not other cell types, are more severely affected by Stag2 loss in the CNS. First, STAG2-cohesin may be more abundant than STAG1-cohesin in postmitotic OLs, making them more dependent on STAG2 for proper functions. Second, STAG1-cohesin preferentially localizes to CTCF-enriched TAD boundaries whereas STAG2-cohesin is more enriched at enhancers lacking CTCF (Kojic et al., 2018). Enhancers are critical for cell-type-specific gene transcriptional programs. To cooperate with the axonal growth during postnatal neurodevelopment, enhancer-enriched transcription factors induce timely and robust gene expression in oligodendrocytes for proper myelination (Mitew et al., 2014). The high demand for enhancer function may render the transcription of myelination genes more reliant on STAG2-cohesin. Finally, the C-terminal regions of STAG1 and STAG2 are divergent in sequence and may bind to different interacting proteins and be subjected to differential regulation. STAG2 may interact with oligodendrocyte-specific transcription factors and be preferentially recruited to myelination genes. It will be interesting to investigate the interactomes of STAG1 and STAG2 in oligodendrocytes using mass spectrometry.
STAG2-mediated chromosome looping and transcription
The mechanisms by which STAG2-dependent chromosome looping facilitates transcription are unclear at present. We propose several models that are not mutually exclusive (Figure 8). First, by forming promoter–enhancer loops, STAG2-cohesin brings the mediator complex and other enhancer-binding factors to the spatial proximity of the general transcriptional machinery at the promoter, thereby enhancing RNA polymerase II recruitment and transcription initiation. The existence of P-stripes at STAG2-dependent genes in the Hi-C maps suggests that STAG2-mediated promoter–enhancer loops may involve enhancers located in the gene body. Second, loop extrusion by STAG2-cohesin may promote transcription elongation by regulating transcription-coupled pre-mRNA processing. For example, STAG2 has been shown to interact with RNA–DNA hybrid structures termed R-loops in vitro and in cells (Pan et al., 2020; Porter et al., 2021). R-loops formed between the nascent pre-mRNA and the DNA template impede transcription elongation and need to be suppressed (Moore and Proudfoot, 2009). When traveling with the transcription machinery on DNA, STAG2-cohesin might directly suppress R-loop formation or recruit other factors, such as the spliceosome, for cotranscriptional pre-mRNA processing and R-loop resolution. Third, STAG2-cohesin may establish promoter–terminator gene loops to recycle the RNA polymerase II that has finished one cycle of transcription back to the TSS for another round of transcription. Future experiments using high-resolution Hi-C methods in oligodendrocytes and ChIP-seq experiments with additional enhancer- and promoter-specific histone marks will allow us to better define the nature of STAG2-dependent promoter-anchored loops and stripes. It will also be interesting to examine whether Stag2 deletion causes the accumulation of R-loops in downregulated genes and the incomplete splicing of their pre-mRNAs.
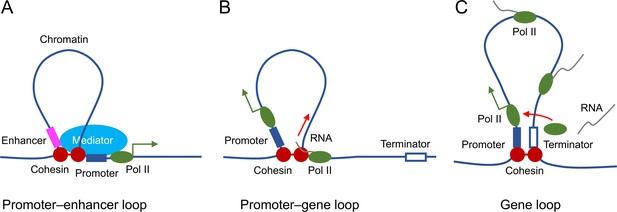
Proposed roles of STAG2-cohesin-mediated loop extrusion during transcription in oligodendrocytes.
(A) STAG2-cohesin-mediated chromosome looping connects the enhancer and the promoter, thus facilitating interactions among oligodendrocyte-specific transcription factors, the mediator complex, and the general transcription machinery including RNA polymerase II. (B) STAG2-cohesin travels along the gene body via transcription-coupled loop extrusion to facilitate pre-mRNA processing. (C) STAG2-cohesin mediates the formation of gene loops that bring the terminator close to the promoter and facilitate Pol II recycling for multiple rounds of transcription.
Conclusion
We have discovered a requirement for the cohesin subunit STAG2 in the myelination of the CNS in mammals. Our findings implicate hypomyelination as a contributing factor to certain phenotypes of cohesinopathy, including growth retardation and neurological disorders. We provide evidence to suggest that STAG2 promotes the myelination transcriptional program in oligodendrocytes through the formation of promoter-anchored loops. Our study establishes oligodendrocytes as a physiologically relevant cell system for dissecting the cellular functions and regulatory mechanisms of cohesin-mediated chromosome folding and genome organization.
Materials and methods
Generation of mouse lines and mouse husbandry
Request a detailed protocolAll animals were handled in accordance with institutional guidelines of the Institutional Animal Care and Use Committee (IACUC; AAALAC unit number 000673) of University of Texas (UT) Southwestern Medical Center under the animal protocol number (APN) 102335. The Stag2 locus was targeted by inserting one neo cassette and two loxP sites flanking exon 8 via homologous recombination in the mouse embryonic stem (ES) cells. G418-selected positive ES clones were screened for successful targeting by nested PCR tests on both 5′ and 3′ integration sites of loxP. Four confirmed ES clones were then microinjected into mouse blastocysts. The chimeras were bred to the R26FLP mouse line for the removal of the neo cassette. Stag2f/+ mice with the 129/B6 background were crossed with Stag2f/y or WT C57BL/6J mice and maintained on this background. For the generation of the inducible system of Stag2f/y;Rosa26CreErt2 mice, Stag2f/f mice were bred to the mouse strain that contains two alleles of the conditional Cre-ERT2 cassette (B6.129-Gt(ROSA)26Sortm1(cre/ERT2)Tyj/J, JAX stock #008463) (Ventura et al., 2007). For the generation of the nervous system-specific Stag2f/y;NesCre mice, the Stag2f/f mice were crossed with the transgenic mice carrying one allele of Cre recombinase driven by the rat nestin promoter and enhancer (Tg(Nes-cre)1Kln, JAX stock #003771) (Giusti et al., 2014; Tronche et al., 1999).
Whole-body knockout mice were generated by CRISPR–Cas9 gene editing technology. Briefly, a pair of guide RNAs (sgRNA; sequences listed in the Key Resource Table) targeting genomic sequence flanking exon 8 of the Stag2 locus were tested for cutting efficiency in cell culture, transcribed in vitro, purified, checked for integrity, and microinjected into B6C3F1 mouse zygotes along with the Cas9 mRNA (5-methylcytidine, pseudouridine, TriLink). 20 ng/μl of Cas9 mRNA and 10 or 20 μg/μl each of sgRNA were used. The injected embryos were transferred to the surrogate mother on the same day. Mosaic F0 founders carrying the Stag2null allele were identified by PCR genotyping. The reduction of the STAG2 protein was confirmed by western blotting in multiple tissues. The F0 founders were crossed with WT C57BL/6J mice to generate the Stag2+/− F1. The mutations in F1 were identified by Sanger Sequencing. Two mouse lines carrying genomic deletions between the Cas9 cleavage sites were chosen for the generation of Stag2null mouse embryos.
All mice were housed in the antigen-free barrier facility with 12 hr light/dark cycles (6 AM on and 6 PM off). Mice were fed a standard rodent chow (2016 Teklad Global 16% protein rodent diet, Harlan Laboratories).
Immunoblotting
Request a detailed protocolThe C-terminal fragment of human STAG2 protein was expressed and purified from Escherichia coli and used as the antigen to generate rabbit polyclonal antibodies against STAG2 at YenZym. Other antibodies were purchased from the following commercial sources: anti-SMC1 (Bethyl Laboratories, A300-055A), anti-SMC3 (Bethyl Laboratories, A300-060A), anti-RAD21 (Bethyl Laboratories, A300-080A), anti-SA1 (Bethyl Laboratories, A302-579A), anti-SA2 (Bethyl Laboratories, A302-581A), anti-α-TUBULIN (Sigma-Aldrich, DM1A), anti-MBP (Abcam, ab7349), anti-PLP1 (Abcam, ab28486), and anti-H3K27ac (Abcam, ab4729).
For immunoblotting, brain hemispheres were homogenized in a Precellys tissue homogenizer (Bertin Instruments) with the lysis buffer [20 mM Tris–HCl (pH 7.7), 137 mM NaCl, 2 mM Ethylenediamine tetraacetic acid (EDTA), 10% (vol/vol) glycerol, 1% (vol/vol) Triton X-100, 0.5 mM dithiothreitol, 1 mM Phenylmethylsulfonyl fluoride (PMSF), 1 mM Na3VO4, 10 mM β-glycerophosphate, 5 mM NaF, and protease inhibitors (Roche)]. Homogenized brain tissues were lysed on ice for 1 hr. The lysate was then subjected to centrifugation at 20,817 × g at 4°C for 20 min and further cleared by filtering through a 0.45 μm filter. The cleared lysate was analyzed by sodium dodecyl sulfate–polyacrylamide gel electrophoresis and transferred to membranes, which was then incubated with the appropriate primary and secondary antibodies. The blots were imaged with the Odyssey Infrared Imaging System (LI-COR).
Tissue histology and immunohistochemistry
Request a detailed protocolMouse brains were fixed in 10% neutral buffered formalin solution for 48 hr followed by paraffin embedding and coronal or sagittal sectioning at 5 μm. H&E staining and LFB staining were performed by the Molecular Pathology Core at UT Southwestern Medical Center. Investigators were blinded to the genotype. Images were acquired with the DM2000 microscope (Leica) at ×1.25 resolution.
Immunohistochemistry was performed as previously described (Choi et al., 2016). Briefly, deparaffinized sections were fixed with 4% paraformaldehyde, subjected to antigen retrieval by boiling with 10 mM sodium citrate (pH 6.0), and then incubated with the indicated antibodies at 1:100 dilution. The slides were scanned with an Axioscan.Z1 microscope (Zeiss) at ×40 resolution at the Whole Brain Microscopy Facility at UT Southwestern Medical Center. Images were processed and quantified with ImageJ. For the myelinated fiber length measurement and coherency analysis, coronal sections of the brain cortex stained with the anti-MBP antibody were processed as previously described (van Tilborg et al., 2017). The myelinated axial thinning and fiber length measurement were performed by the plugin DiameterJ. The coherency analysis of myelinated axons was performed with the plugin OrientationJ.
Isolation of primary oligodendrocytes
Request a detailed protocolThe immunomagnetic isolation of oligodendrocytes from Stag2f/y and Stag2f/y;NesCre P12-P14 pups was conducted using anti-O4 microbeads (Miltenyi Biotec) according to a published protocol (Flores-Obando et al., 2018). Briefly, brain cortices were dissected, pooled, minced into 1 mm3 cubes, and incubated with the Papain dissociation solution (neurobasal medium with 1% penicillin–streptomycin, 1% L-glutamine, 2% B27 supplement, 20–30 U/ml of Papain, and 2500 U DNase I) in a 37°C, 5% CO2 incubator for more than 20 min. The enzymatic digestion was inactivated by the addition of 1 ml of fetal bovine serum (FBS). Gentle trituration by 10 ml, 5 ml and 1-ml pipettes was applied to break up cell clumps. Cells were collected by centrifugation (200 × g, 10 min), washed first with serum-containing medium (neurobasal medium with 1% penicillin–streptomycin, 1% L-glutamine, 2% B27 supplement, and 10% FBS), and then with the magnetic cell sorting (MCS) buffer (phosphate-buffered saline, pH 7.2, with 0.5% bovine serum albumin [BSA], 0.5 mM EDTA, 5 μg/ml insulin and 1 g/l glucose). The cell pellet was resuspended in the MCS buffer and incubated with anti-O4 microbeads at 10 μl/107 cells at 4°C for 15 min followed by 1× wash with the MCS buffer. The O4+ immature oligodendrocytes were sorted through the magnetic LS columns according to the manufacturer’s instruction. Freshly prepared oligodendrocytes were directly used or fixed for subsequent analysis.
Metabolic cage analysis
Request a detailed protocolMice were singly housed in shoebox-sized cages with a 5-day acclimation period followed with a 4-day recording period. Recorded parameters were analyzed by the TSE system and normalized to body weight. The experiments were conducted by the core personnel under the core protocol at the Metabolic Phenotyping Core at UT Southwestern Medical Center. Investigators were blinded to the genotype.
Growth hormone and IGF-1 detection
Request a detailed protocolBlood samples were collected from facial bleeding without fasting. Plasma growth hormone levels were determined with the rat/mouse growth hormone ELISA kit (EMD Milipore, EZRMGH-45K). Plasma IGF-1 concentrations were measured using the mouse/rat IGF1 Quantikine ELISA kit (R&D Systems).
Sterol and oxysterol composition analysis
Request a detailed protocolBrain hemispheres were preweighed and snap-frozen for extraction and measurement by mass spectrometry. The sterol extraction and quantitative analysis were conducted at the Center of Human Nutrition at UT Southwestern Medical Center as described previously (McDonald et al., 2012).
Electron microscopy
Request a detailed protocolStag2f/y and Stag2f/y;NesCre P18 pups were transcardially perfused with 4% paraformaldehyde, 1% glutaraldehyde in 0.1 M sodium cacodylate buffer (pH 7.4). Tissues were dissected and fixed with 2.5% (vol/vol) glutaraldehyde in 0.1 M sodium cacodylate buffer (pH 7.4) for at least 2 hr. After three rinses with the 0.1 M sodium cacodylate buffer, optic nerve samples were embedded in 3% agarose and sliced into small blocks. All samples were again rinsed with the 0.1 M sodium cacodylate buffer three times and postfixed with 1% osmium tetroxide and 0.8% potassium ferricyanide in the 0.1 M sodium cacodylate buffer for 3 hr at room temperature. Blocks were rinsed with water and en bloc stained with 4% uranyl acetate in 50% ethanol for 2 hr. Samples were dehydrated with increasing concentrations of ethanol, transitioned into propylene oxide, infiltrated with Embed-812 resin, and polymerized in a 60°C oven overnight. Blocks were sectioned with a diamond knife (Diatome) on a Leica Ultracut 7 ultramicrotome (Leica Microsystems) and collected onto copper grids, poststained with 2% aqueous uranyl acetate and lead citrate. Images were acquired on a Tecnai G2 Spirit transmission electron microscope (Thermo Fisher) equipped with a LaB6 source using a voltage of 120 kV. Tissue processing, sectioning, and staining were completed by the Electron Microscopy Core at UT Southwestern Medical Center.
RNA-seq library preparation and sequencing
Request a detailed protocolTotal RNA was extracted from brain hemispheres or isolated oligodendrocytes with Trizol. RNA integrity was determined by the Agilent BioAnalyzer 2100. TruSeq Stranded mRNA library prep kit (Illumina) was used to generate the mRNA libraries. The libraries were analyzed by the Bioanalyzer and multiplexed and sequenced using the NextSeq 500 high output kit (400 M reads) for the brain libraries or NextSeq 500 mid output kit (130 M reads) for the isolated oligodendrocytes libraries at the Next Generation Sequencing Core at UT Southwestern Medical Center.
Differential expression and pathway analysis
Request a detailed protocolRaw data from the sequencer were demultiplexed and converted to fastq files using bcl2fastq (v2.17, Illumina). The fastq files were checked for quality using fastqc (v0.11.2) Andrews, 2010 and fastq_screen (v0.4.4) (Wingett, 2011). Fastq files were mapped to the mm10 mouse reference genome (from iGenomes) using STAR (Dobin et al., 2013). Read counts were then generated using featureCounts (Liao et al., 2014). TMM normalization and differential expression analysis were performed using edgeR (Robinson et al., 2010). Pathway analysis was performed with the IPA software. Genes with more than 1.5-fold change and false-discovery rate FDR <0.01 were included in the brain RNA-seq pathway analysis. Genes with more than twofold change and FDR <0.05 were used for the pathway analysis of the RNA-seq data from oligodendrocytes.
RT-qPCR analysis
Request a detailed protocolSingle-stranded cDNAs were converted from 2 µg of total RNA extracted from mouse brains with the high-capacity cDNA reverse transcription kit (Applied Biosystems). Quantitative PCR was conducted to determine transcript levels using gene-specific TaqMan probes (Applied Biosystems).
Single-cell RNA-seq
Request a detailed protocolSingle-cell suspension was prepared from forebrains of P13 Stag2f/y or Stag2f/y;NesCre pups using the Papain Dissociation System (Worthington Biochemical, LK003150) according to the manufacturer’s instructions. Biological duplicates were made for each genotype. Single-cell RNA-seq libraries were generated with the Chromium Single Cell 3′ GEM, Library & Gel Bead Kit v3 (10× Genomics) according to the manufacturer’s guidelines. Cell density and viability were checked by the TC-20 Cell Counter (Bio-Rad). Cells were then loaded onto Chip B in the Chromium Controller (10× Genomics). 10,000 cells were targeted for each sample. The libraries were analyzed by the Bioanalyzer (Agilent) and pair-end sequenced in two flowcells of the NextSeq 500 High Output (400 M) run. The sequencing was performed at the Next Generation Sequencing Core at UT Southwestern Medical Center.
Data demultiplexing and alignment were performed using the Cell Ranger pipeline (https://support.10xgenomics.com/single-cell-gene-expression/software/pipelines/latest/using/ mkfastq) (10× Genomics). The raw features, barcodes, and matrixes were used as input for further analysis using the R package Seurat3 (Butler et al., 2018; Stuart et al., 2019) (https://satijalab.org/seurat/). Cells were filtered by the following criteria: nFeature_RNA (200–9500) and percent.mt <10. After filtering, a total of 5834 cells in Stag2f/y#1, 4699 cells in Stag2f/y#2, 9050 cells in Stag2f/y;NesCre#1, and 3073 cells in Stag2f/y;NesCre#2 were used for downstream analysis. 2000 variable features were found from each normalized dataset. All datasets were then integrated using identified anchors (dims = 1:30). Standard scaling and principal component analysis, clustering (resolution = 0.5), and tSNE reduction (dims = 1:30) were performed on the integrated dataset. Cluster biomarkers were identified, and top features were examined. Clusters were then manually assigned to distinct cell-type identities with knowledge from previous studies (Cahoy et al., 2008; Dulken et al., 2019; Marques et al., 2018; Marques et al., 2016; Marton et al., 2019; Saunders et al., 2018; Zeisel et al., 2018; Zywitza et al., 2018) (http://www.brainrnaseq.org/) (http://dropviz.org/). Clusters with the same cell-type identities were merged. Five clusters of oligodendrocyte lineage [cycling oligodendrocyte progenitors (OPCcycs), oligodendrocyte progenitors (OPCs), newly formed oligodendrocytes (NFOLs), myelin-forming oligodendrocytes (mFOLs), and fully matured oligodendrocytes (MFOLs)] were identified and selected for indicated gene expression comparison and plotting using Vlnplot or FeaturePlot functions. The trajectory analysis was performed using Monocle3 (Cao et al., 2019) in the oligodendrocyte cell population. Gene density plot over pseudotime was generated as previously described (Luecken and Theis, 2019).
ChIP-seq
Request a detailed protocolChromatin immunoprecipitation (ChIP) was performed as previously described (Liu et al., 2017). Briefly, isolated oligodendrocytes were fixed with 1% formaldehyde and fragmented with a sonicator (Branson 450). The fragmented chromatin was incubated with antibodies overnight at 4°C. Dynabeads Protein A (Thermo Fisher Scientific) was used for the immunoprecipitation. Libraries were generated by the Next Gen DNA Library Kit (Active Motif) with the Next Gen Indexing Kit (Active Motif) for STAG2 ChIP-seq or the KAPA HyperPrep Kits (KAPA Systems) for histone ChIP-seq. The libraries were analyzed by the Bioanalyzer and pool sequenced with the NextSeq 500 mid output (130 M) kit. After mapping reads to the mouse genome (mm10) by bowtie2 (v2.2.3) (Langmead and Salzberg, 2012) with the parameter ‘–sensitive’, we performed filtering by removing alignments with mapping quality less than 10 and then removing duplicate reads identified by Picard MarkDuplicates (v1.127). For STAG2 ChIP-seq, Picard MarkDuplicate was used to remove duplicates together with options to use molecular identifiers (MIDs) information in the reads. Enriched regions (peaks) were identified using MACS2 (v2.0.10) (Zhang et al., 2008), with a q-value cutoff of 0.05 for peaks. Peak regions were annotated by HOMER (Ross-Innes et al., 2012).
Hi-C library generation, sequencing, and analysis
Request a detailed protocolHi-C was performed at the Genome Technology Center at NYU Langone Health from 3.5 to 4.0 μg of DNA isolated from cells cross-linked with 2% formaldehyde at room temperature for 10 min. Experiments were performed in duplicates following the instructions from the Arima Hi-C kit (Arima Genomics, San Diego, CA). Subsequently, Illumina-compatible sequencing libraries were prepared by using a modified version of the KAPA HyperPrep library kit (KAPA BioSystems, Willmington, MA). Quality check steps were performed to assess the fraction of proximally ligated DNA labeled with biotin, and the optimal number of PCR reactions needed to make libraries. The libraries were loaded into an Illumina flowcell (Illumina, San Diego, CA) on a NovaSeq 6000 instrument for paired-end 50 reads.
Hi-C analysis was performed using the HiC-Bench pipeline (Lazaris et al., 2017; Tsirigos et al., 2012) (https://github.com/NYU-BFX/hic-bench) and HiC-Pro v3.1.0 (Servant et al., 2015). The read pairs were aligned and filtered with the following parameters: Genome-build=mm10; –very-sensitive-local –local; mapq = 20; –min-dist 25000 –max-offset 500. The Juicer ‘pre’ tool (Durand et al., 2016) (RRID: SCR_017226, v1.11.09; https://github.com/aidenlab/juicer) was used to generate the.hic file with default parameters. Sample duplicates were combined. The compartment analysis was done using the HOMER tool (Heinz et al., 2010) (http://homer.ucsd.edu/homer/index.html) with 100 kb bins. H3K27ac ChIP-seq data were used to assign A/B compartments. Eigenvector-1 bins were considered shifted (AB and BA) when the bin sign changed and the delta value was greater than 1.5. TADs and boundaries were identified at 40 kb resolution with the HiCRatio method with the follow parameters: –min-lambda=0.0 –max-lambda=1.0 –n-lambda=6 –gamma = 0 –distance = 500 kb –fdr = 0.1. TADs were also identified using the Juicer tools (v1.22.01) arrowhead at 10 and 25 kb resolution. Aggregate TAD analysis was performed on TAD boundaries by coolpup.py (Flyamer et al., 2020) or GENOVA (van der Weide et al., 2021). The.hic files were converted to.cool format for visualization and plotting with pyGenomeTracks (Lopez-Delisle et al., 2021) at 5 kb resolution.
Loop analysis and RNA-seq integration
Request a detailed protocolThe loops were classified into group-specific loops and common loops by using the significance cutoffs provided by Fit-HiC (Ay et al., 2014). A q-value cutoff of 0.01 was used to identify significant loops in both groups. A loop is considered ‘group-specific’ if it is only present in one group with a q value <0.01 and not present in the other group with cutoff of q val <0.1. Loop anchors were annotated with the gene promoter information (promoter defined as ±2 kb from the TSS). The genes were classified into ‘down’ and ‘up’ regulated genes using an FDR cutoff of 0.05, logFC cutoff of ±0.58 and logCPM >0. ‘stable’ or less changed genes are defined as logFC <0.38, and logCPM >0. Random 1000 genes were chosen for analysis and plotting. The active genes (logCPM >0) were also grouped in ‘high’, ‘mid’, and ‘low’ expression groups by separating the genes in three quantiles according to the logCPM values. For the loop enrichment scores, normalized contact scores were computed using Fit-HiC at 10 kb resolution and bias corrected. Pile-up analysis was performed with coolpup.py (Flyamer et al., 2020) with the KR method to balance the weight and random shift controls for distance normalization at 5 kb or using GENOVA.
Appendix 1
Reagent type (species) or resource | Designation | Source or reference | Identifiers | Additional information |
---|---|---|---|---|
Strain, strain background (Mus musculus, female) | Stag2+/− | This paper | Exon8 of Stag2 was targeted by CRISPR–Cas9 (see Materials and methods) | |
Strain, strain background (Mus. musculus, both sex) | Stag2f/y; Stag2f/f | This paper | Exon8 of Stag2 genomic locus was flanked by loxP sites (see Materials and methods) | |
Strain, strain background (Mus. musculus, both sex) | C57BL/6J | The Jackson Laboratory | 000664; RRID:IMSR_JAX:000664 | |
Strain, strain background (Mus. musculus, both sex) | B6.129-Gt(ROSA)26 Sortm1(cre/ERT2)Tyj/J | The Jackson Laboratory | 008463; RRID:IMSR_JAX:008463 | |
Strain, strain background (Mus. musculus, male) | B6.Cg-Tg(Nes-cre)1Kln/J | The Jackson Laboratory | 003771; RRID:IMSR_JAX:003771 | |
Antibody | anti-STAG2 (Rabbit polyclonal) | This paper | The C-terminus recombinant protein of STAG2 (Homo sapiens) was used to generate the antibody; WB (1:1000) | |
Antibody | anti-α-TUBULIN (Mouse monoclonal) | Sigma-Aldrich | T9026; RRID:AB_477593 | WB (1:1000) |
Antibody | anti-SA1 (Rabbit polyclonal) | Bethyl Laboratories | A302-579A; RRID:AB_2034857 | WB (1:1000) |
Antibody | anti-SMC1 (Rabbit polyclonal) | Bethyl Laboratories | A300-055A RRID:AB_2192467 | WB (1:1000) |
Antibody | anti-SMC3 (Rabbit polyclonal) | Bethyl Laboratories | A300-060A; RRID:AB_67579 | WB (1:1000) |
Antibody | anti-RAD21 (Rabbit polyclonal) | Bethyl Laboratories | A300-080a; RRID:AB_2176615 | WB (1:1000) |
Antibody | anti-MBP (Rat monoclonal) | Abcam | ab7349; RRID:AB_305869 | IHC (1:100) |
Antibody | anti-PLP1 (Rabbit polyclonal) | Abcam | ab28486; RRID:AB_776593 | IHC (1:100) |
Antibody | anti-GFAP (Rabbit polyclonal) | Abcam | ab7260; RRID:AB_305808 | IHC (1:100) |
Antibody | anti-MAP2 (Rabbit polyclonal) | Abcam | ab32454; RRID:AB_776174 | IHC (1:50) |
Antibody | anti-H3K27ac (Rabbit polyclonal) | Abcam | ab4729; RRID:AB_2118291 | ChIP (5 μl per test) |
Antibody | anti-O4 Microbeads (Mouse monoclonal) | Miltenyi Biotec | 130-094-543; RRID:AB_2847907 | MACS (10 μl per 107 cells) |
Antibody | anti-rabbit IgG (H+L), DyLight 800 Conjugate (Goat polyclonal) | Cell Signaling Technology | 5151 S; RRID:AB_10697505 | WB (1:5000) |
Antibody | anti-mouse IgG (H+L), DyLight 680 Conjugate (Goat polyclonal) | Cell Signaling Technology | 5470 S; AB_10696895 | WB (1:5000) |
Antibody | anti-rat IgG (H+L), Alexa Fluor 568 (Goat polyclonal) | Thermo Fisher Scientific | A-11077; RRID:AB_2534121 | IHC (1:500) |
Antibody | anti-rabbit IgG (H+L), Alexa Fluor 488 (Goat polyclonal) | Thermo Fisher Scientific | A-11008; RRID:AB_143165 | IHC(1:500) |
Sequence-based reagent | sgRNA#1 target on Stag2 | This paper | CRISPR single-guide RNA target sequence | Target sequence: TAGCCAACCTCTTTCTCTATTGG |
Sequence-based reagent | sgRNA#2 target on Stag2 | This paper | CRISPR single-guide RNA target sequence | Target sequence: CAGACAGTATACTGTAATGGAGG |
Sequence-based reagent | TaqMan probes: Stag2 | Thermo Fisher Scientific | Mm01311611_m1 | |
Sequence-based reagent | TaqMan probes: Klk6 | Thermo Fisher Scientific | Mm00478322_m1 | |
Sequence-based reagent | TaqMan probes: Ninj2 | Thermo Fisher Scientific | Mm00450216_m1 | |
Sequence-based reagent | TaqMan probes: Cpm | Thermo Fisher Scientific | Mm01250802_m1 | |
Sequence-based reagent | TaqMan probes: Fa2h | Thermo Fisher Scientific | Mm00626259_m1 | |
Sequence-based reagent | TaqMan probes: Gapdh | Thermo Fisher Scientific | Mm99999915_g1 | |
Sequence-based reagent | Stag2 gt 5 F | This paper | Genotype sequence primers | GGTATTTACTTGATAGCCAACC |
Sequence-based reagent | Stag2 gt 5 R | This paper | Genotype sequence primers | CTCATCTTGATTTTCCTGAAGC |
Sequence-based reagent | Stag2 gt 3 F | This paper | Genotype sequence primers | GGTTGAGACAGACAGTATAC |
Sequence-based reagent | Stag2 gt 3 R | This paper | Genotype sequence primers | AGGCTGGACTATGACAACTC |
Sequence-based reagent | ISH Probe Stag2 P1 F | This paper | Riboprobe synthesis primers | TACGGTACCGACCTTTCAGATGTCACTCCG |
Sequence-based reagent | ISH Probe Stag2 P1 R | This paper | Riboprobe synthesis primers | GAAGGATCCGCATCGGATAGACACTCATGA |
Sequence-based reagent | ISH Probe Stag2 P2 F | This paper | Riboprobe synthesis primers | TACGGATCCGACCTTTCAGATGTCACTCCG |
Sequence-based reagent | ISH Probe Stag2 P2 R | This paper | Riboprobe synthesis primers | GAAGGTACCGCATCGGATAGACACTCATGA |
Sequence-based reagent | ISH Probe Stag1 P1 F | This paper | Riboprobe synthesis primers | TTAGGTACCTTACAATGCCTGGTCCTCAGT |
Sequence-based reagent | ISH Probe Stag1 P1 R | This paper | Riboprobe synthesis primers | GAAGGATCCCTTTCATTGGCTCTCTTCCC |
Sequence-based reagent | ISH Probe Stag1 P2 F | This paper | Riboprobe synthesis primers | TTAGGATCCTTACAATGCCTGGTCCTCAGT |
Sequence-based reagent | ISH Probe Stag1 P2 R | This paper | Riboprobe synthesis primers | GAAGGTACCCTTTCATTGGCTCTCTTCCC |
Commercial assay or kit | Arima-HiC Kit | Arima Genomics | 510008 | |
Chemical compound, drug | Tamoxifen | Sigma-Aldrich | T5648 | |
Chemical compound, drug | 4-Hydroxytamoxifen | Sigma-Aldrich | H7904 | |
Software, algorithm | GraphPad Prism | GraphPad Software | RRID:SCR_002798; https://www.graphpad.com/scientific-software/prism/ | |
Software, algorithm | ImageJ (Fiji) | ImageJ | RRID:SCR_002285; https://imagej.net/software/fiji/ | |
Software, algorithm | RStudio | The R Foundation | RRID:SCR_000432; https://www.rstudio.com/ | |
Software, algorithm | Bcl2fastq | Illumina | RRID:SCR_015058 | v2.17 |
Software, algorithm | Fastqc | Andrews, 2010; PMID:24501021 | RRID:SCR_014583 | v0.11.2 |
Software, algorithm | Fastq_screen | Wingett, 2011 | RRID:SCR_000141; https://www.bioinformatics.babraham.ac.uk/projects/fastqc/ | v0.4.4 |
Software, algorithm | STAR | Dobin et al., 2013; PMID:23104886 | RRID:SCR_004463; https://github.com/alexdobin/STAR | v2.5.3a |
Software, algorithm | FeatureCounts | Liao et al., 2014; PMID:24227677 | RRID:SCR_012919; https://bioconductor.org/packages/release/bioc/html/Rsubread.html | |
Software, algorithm | edgeR | Robinson et al., 2010; PMID:19910308 | RRID:SCR_012802; https://bioconductor.org/packages/release/bioc/html/edgeR.html | |
Software, algorithm | Ingenuity pathway analysis | QIAGEN, Krämer et al., 2014; PMID:24336805 | RRID:SCR_008653; https://www.qiagenbioinformatics.com/products/ingenuity-pathway-analysis | |
Software, algorithm | MACS2 | Zhang et al., 2008; PMID:18798982 | RRID:SCR_013291 | v2.0.10 |
Software, algorithm | Bowtie2 | Langmead and Salzberg, 2012; PMID:22388286 | RRID:SCR_016368 | v2.2.3 |
Software, algorithm | Picard MarkDuplicates | Broad Institute, GitHub Repository | RRID:SCR_006525; http://broadinstitute.github.io/picard/ | v1.127 |
Software, algorithm | HOMER | Heinz et al., 2010, Ross-Innes et al., 2012; PMID:20513432 | RRID:SCR_010881; http://homer.ucsd.edu/homer/ | |
Software, algorithm | Deeptools | Ramírez et al., 2016; PMID:27079975 | RRID:SCR_016366; https://deeptools.readthedocs.io/en/develop/ | |
Software, algorithm | Galaxy | Afgan et al., 2018; PMID:29790989 | RRID:SCR_006281; https://usegalaxy.org | |
Software, algorithm | Cell Ranger | 10× Genomics | RRID:SCR_017344; https://support.10xgenomics.com/single-cell-gene-expression/software/pipelines/latest/using/mkfastq | |
Software, algorithm | Seurat | New York Genome Center; Stuart et al., 2019; PMID:31178118 | RRID:SCR_016341; https://satijalab.org/seurat | Satija Lab |
Software, algorithm | Monocle3 | UW Genome Sciences; Cao et al., 2019; PMID:30787437 | RRID:SCR_018685; https://cole-trapnell-lab.github.io/monocle3/ | Cole Trapnell’s Lab, v3.0 |
Software, algorithm | HiC-Bench pipeline | Lazaris et al., 2017, Tsirigos et al., 2012; PMID:22113082 | https://github.com/NYU-BFX/hic-bench | v0.1 |
Software, algorithm | Juicer ‘pre’ tool | Durand et al., 2016; PMID:27467249 | RRID:SCR_017226; https://github.com/aidenlab/juicer | Aiden Lab, v1.11.09 |
Software, algorithm | Juicebox | Aiden Lab, BCM | RRID:SCR_021172; https://github.com/aidenlab/Juicebox | v1.5.1 |
Software, algorithm | Hic2cool | Abdennur and Mirny, 2020; PMID:31290943 | https://github.com/4dn-dcic/hic2cool | v0.8.3 |
Software, algorithm | pyGenomeTracks | Lopez-Delisle et al., 2021; PMID:32745185 | https://github.com/deeptools/pyGenomeTracks | v3.7 |
Software, algorithm | Fit-HiC | Ay et al., 2014; PMID:24501021 | https://github.com/ay-lab/fithic | v2.0.7 |
Software, algorithm | Coolpup.py | Flyamer et al., 2020; PMID:32003791 | https://github.com/open2c/coolpuppy | v0.9.5 |
Software, algorithm | clusterProfiler | Bioinformatics Group, Southern Medical University; Wu et al., 2021; PMID:34557778 | RRID:SCR_016884; https://github.com/YuLab-SMU/clusterProfiler | v4.4.1 |
Software, algorithm | HiC-Pro | Servant et al., 2015; PMID:26619908 | RRID:SCR_017643 | v3.1.0 |
Software, algorithm | HiCRep | Yang et al., 2017; PMID:28855260 | https://github.com/TaoYang-dev/hicrep | v1.11.0 |
Software, algorithm | GENOVA | van der Weide et al., 2021; PMID:34046591 | https://github.com/robinweide/GENOVA | v1.0.0.9 |
Data availability
The RNA-seq, scRNA-seq, ChIP-seq, and Hi-C datasets generated and analyzed during the current study are available in the GEO repository, with the accession number GSE186894.
-
NCBI Gene Expression OmnibusID GSE186894. STAG2 promotes the myelination transcriptional program in oligodendrocytes.
References
-
The galaxy platform for accessible, reproducible and collaborative biomedical analyses: 2018 updateNucleic Acids Research 46:W537–W544.https://doi.org/10.1093/nar/gky379
-
Cohesin mutations in cancerCold Spring Harbor Perspectives in Medicine 6:a026476.https://doi.org/10.1101/cshperspect.a026476
-
STAR: ultrafast universal RNA-seq alignerBioinformatics 29:15–21.https://doi.org/10.1093/bioinformatics/bts635
-
Rapid and specific immunomagnetic isolation of mouse primary oligodendrocytesJournal of Visualized Experiments 5:57543.https://doi.org/10.3791/57543
-
Coolpup.py: versatile pile-up analysis of hi-C dataBioinformatics 36:2980–2985.https://doi.org/10.1093/bioinformatics/btaa073
-
Behavioral phenotyping of nestin-cre mice: implications for genetic mouse models of psychiatric disordersJournal of Psychiatric Research 55:87–95.https://doi.org/10.1016/j.jpsychires.2014.04.002
-
Structure of cohesin subcomplex pinpoints direct shugoshin-wapl antagonism in centromeric cohesionNature Structural & Molecular Biology 21:864–870.https://doi.org/10.1038/nsmb.2880
-
Human cohesin compacts DNA by loop extrusionScience 366:1345–1349.https://doi.org/10.1126/science.aaz4475
-
Distinct roles of cohesin-SA1 and cohesin-SA2 in 3D chromosome organizationNature Structural & Molecular Biology 25:496–504.https://doi.org/10.1038/s41594-018-0070-4
-
Fast gapped-read alignment with bowtie 2Nature Methods 9:357–359.https://doi.org/10.1038/nmeth.1923
-
Current best practices in single-cell RNA-seq analysis: a tutorialMolecular Systems Biology 15:e8746.https://doi.org/10.15252/msb.20188746
-
Somatic mutation in cancer and normal cellsScience 349:1483–1489.https://doi.org/10.1126/science.aab4082
-
A comprehensive method for extraction and quantitative analysis of sterols and secosteroids from human plasmaJournal of Lipid Research 53:1399–1409.https://doi.org/10.1194/jlr.D022285
-
Cohesin SA1 and SA2 are RNA binding proteins that localize to RNA containing regions on DNANucleic Acids Research 48:5639–5655.https://doi.org/10.1093/nar/gkaa284
-
DeepTools2: a next generation web server for deep-sequencing data analysisNucleic Acids Research 44:W160–W165.https://doi.org/10.1093/nar/gkw257
-
High cholesterol level is essential for myelin membrane growthNature Neuroscience 8:468–475.https://doi.org/10.1038/nn1426
-
The transcriptome of mouse central nervous system myelinScientific Reports 6:25828.https://doi.org/10.1038/srep25828
-
SMC complexes: from DNA to chromosomesNature Reviews. Molecular Cell Biology 17:399–412.https://doi.org/10.1038/nrm.2016.30
-
Hi-C analyses with GENOVA: a case study with cohesin variantsNAR Genomics and Bioinformatics 3:lqab040.https://doi.org/10.1093/nargab/lqab040
-
Neuropathological analysis of an adult case of the cornelia de lange syndromeActa Neuropathologica 104:327–332.https://doi.org/10.1007/s00401-002-0562-4
-
Myelin dynamics throughout life: an ever-changing landscape?Frontiers in Cellular Neuroscience 12:424.https://doi.org/10.3389/fncel.2018.00424
-
Hypomyelinating leukodystrophies - unravelling myelin biologyNature Reviews. Neurology 17:88–103.https://doi.org/10.1038/s41582-020-00432-1
-
Organization of chromosomal DNA by SMC complexesAnnual Review of Genetics 53:445–482.https://doi.org/10.1146/annurev-genet-112618-043633
-
Model-based analysis of chip-seq (MACS)Genome Biology 9:R137.https://doi.org/10.1186/gb-2008-9-9-r137
-
Regulation of sister chromatid cohesion during the mitotic cell cycleScience China. Life Sciences 58:1089–1098.https://doi.org/10.1007/s11427-015-4956-7
Article and author information
Author details
Funding
National Natural Science Foundation of China (Project 32130053)
- Hongtao Yu
National Institutes of Health (1R01GM124096)
- Hongtao Yu
Cancer Prevention and Research Institute of Texas (RP160667-P2)
- Hongtao Yu
Welch Foundation (I-1441)
- Hongtao Yu
The funders had no role in study design, data collection, and interpretation, or the decision to submit the work for publication.
Acknowledgements
We thank Sung Jun Bae for taking the mouse photos and John Shelton for help with histology and in situ hybridization. We are grateful to Jeffrey McDonald for the sterol composition analysis, Richard Lu and Lu Sun for providing reagents and advice for the isolation of oligodendrocytes, and Applied Bioinformatics Laboratories at NYU Langone Health for the Hi-C analysis. We also thank the Yu lab members for helpful discussions and for reading the manuscript critically. This study was supported by the National Natural Science Foundation of China (Project 32130053), the U.S. National Institutes of Health (1R01GM124096), the Cancer Prevention and Research Institute of Texas (CPRIT) (RP160667-P2), and the Welch foundation (I-1441).
Ethics
All animals were handled in accordance with institutional guidelines of the Institutional Animal Care and Use Committee (IACUC; AAALAC unit number 000673) of University of Texas (UT) Southwestern Medical Center under the animal protocol number (APN) 102335.
Copyright
© 2022, Cheng et al.
This article is distributed under the terms of the Creative Commons Attribution License, which permits unrestricted use and redistribution provided that the original author and source are credited.
Metrics
-
- 1,702
- views
-
- 364
- downloads
-
- 13
- citations
Views, downloads and citations are aggregated across all versions of this paper published by eLife.
Download links
Downloads (link to download the article as PDF)
Open citations (links to open the citations from this article in various online reference manager services)
Cite this article (links to download the citations from this article in formats compatible with various reference manager tools)
Further reading
-
- Cell Biology
- Immunology and Inflammation
Macrophages are crucial in the body’s inflammatory response, with tightly regulated functions for optimal immune system performance. Our study reveals that the RAS–p110α signalling pathway, known for its involvement in various biological processes and tumourigenesis, regulates two vital aspects of the inflammatory response in macrophages: the initial monocyte movement and later-stage lysosomal function. Disrupting this pathway, either in a mouse model or through drug intervention, hampers the inflammatory response, leading to delayed resolution and the development of more severe acute inflammatory reactions in live models. This discovery uncovers a previously unknown role of the p110α isoform in immune regulation within macrophages, offering insight into the complex mechanisms governing their function during inflammation and opening new avenues for modulating inflammatory responses.
-
- Cell Biology
G protein-coupled receptors (GPCRs) are integral membrane proteins which closely interact with their plasma membrane lipid microenvironment. Cholesterol is a lipid enriched at the plasma membrane with pivotal roles in the control of membrane fluidity and maintenance of membrane microarchitecture, directly impacting on GPCR stability, dynamics, and function. Cholesterol extraction from pancreatic beta cells has previously been shown to disrupt the internalisation, clustering, and cAMP responses of the glucagon-like peptide-1 receptor (GLP-1R), a class B1 GPCR with key roles in the control of blood glucose levels via the potentiation of insulin secretion in beta cells and weight reduction via the modulation of brain appetite control centres. Here, we unveil the detrimental effect of a high cholesterol diet on GLP-1R-dependent glucoregulation in vivo, and the improvement in GLP-1R function that a reduction in cholesterol synthesis using simvastatin exerts in pancreatic islets. We next identify and map sites of cholesterol high occupancy and residence time on active vs inactive GLP-1Rs using coarse-grained molecular dynamics (cgMD) simulations, followed by a screen of key residues selected from these sites and detailed analyses of the effects of mutating one of these, Val229, to alanine on GLP-1R-cholesterol interactions, plasma membrane behaviours, clustering, trafficking and signalling in INS-1 832/3 rat pancreatic beta cells and primary mouse islets, unveiling an improved insulin secretion profile for the V229A mutant receptor. This study (1) highlights the role of cholesterol in regulating GLP-1R responses in vivo; (2) provides a detailed map of GLP-1R - cholesterol binding sites in model membranes; (3) validates their functional relevance in beta cells; and (4) highlights their potential as locations for the rational design of novel allosteric modulators with the capacity to fine-tune GLP-1R responses.