Orai-mediated calcium entry determines activity of central dopaminergic neurons by regulation of gene expression
eLife assessment
In Drosophila melanogaster, the SOCE channel Orai is required for the development of flight-promoting dopaminergic neurons. The Hasan laboratory has previously shown that disabling Orai function impairs Drosophila flight due to aberrant neuronal development at the pupal stage. In this fundamental study, Mitra et al. show that SOCE drives a transcriptional feedback loop via the homeobox transcription factor, 'Trithorax-like' (Trl), and histone modifiers, Set2 and E(z), to regulate the expression of key genes required for the function of dopaminergic flight neurons, including the muscarinic acetylcholine receptor and the inositol 1,4,5-trisphosphate receptor. This solid study is carefully performed with validated methodology and most of the analyses are rigorous.
https://doi.org/10.7554/eLife.88808.4.sa0Fundamental: Findings that substantially advance our understanding of major research questions
- Landmark
- Fundamental
- Important
- Valuable
- Useful
Solid: Methods, data and analyses broadly support the claims with only minor weaknesses
- Exceptional
- Compelling
- Convincing
- Solid
- Incomplete
- Inadequate
During the peer-review process the editor and reviewers write an eLife Assessment that summarises the significance of the findings reported in the article (on a scale ranging from landmark to useful) and the strength of the evidence (on a scale ranging from exceptional to inadequate). Learn more about eLife Assessments
Abstract
Maturation and fine-tuning of neural circuits frequently require neuromodulatory signals that set the excitability threshold, neuronal connectivity, and synaptic strength. Here, we present a mechanistic study of how neuromodulator-stimulated intracellular Ca2+ signals, through the store-operated Ca2+ channel Orai, regulate intrinsic neuronal properties by control of developmental gene expression in flight-promoting central dopaminergic neurons (fpDANs). The fpDANs receive cholinergic inputs for release of dopamine at a central brain tripartite synapse that sustains flight (Sharma and Hasan, 2020). Cholinergic inputs act on the muscarinic acetylcholine receptor to stimulate intracellular Ca2+ release through the endoplasmic reticulum (ER) localised inositol 1,4,5-trisphosphate receptor followed by ER-store depletion and Orai-mediated store-operated Ca2+ entry (SOCE). Analysis of gene expression in fpDANs followed by genetic, cellular, and molecular studies identified Orai-mediated Ca2+ entry as a key regulator of excitability in fpDANs during circuit maturation. SOCE activates the transcription factor trithorax-like (Trl), which in turn drives expression of a set of genes, including Set2, that encodes a histone 3 lysine 36 methyltransferase (H3K36me3). Set2 function establishes a positive feedback loop, essential for receiving neuromodulatory cholinergic inputs and sustaining SOCE. Chromatin-modifying activity of Set2 changes the epigenetic status of fpDANs and drives expression of key ion channel and signalling genes that determine fpDAN activity. Loss of activity reduces the axonal arborisation of fpDANs within the MB lobe and prevents dopamine release required for the maintenance of long flight.
Introduction
Neural circuitry underlying mature adult behaviours emerges from a combination of developmental gene expression programs and experience-dependent neuronal activity. Holometabolous insects such as Drosophila reconfigure their nervous system during metamorphosis to generate circuitry capable of supporting adult behaviours (Levine, 1984). During pupal development, neurons undergo maturation of their electrical properties with a gradual increase in depolarising responses and consequent synaptic transmission (Hardie et al., 1993; Järvilehto and Finell, 1983). Apart from voltage-gated Ca2+ channels and fast-acting neurotransmitters specific to ionotropic receptors, the developing nervous system also uses neuromodulators, which target metabotropic receptors, show slower response kinetics, and are capable of affecting a larger subset of neurons by means of diffusion-aided volumetric transmission (Taber and Hurley, 2014). Although neuromodulators alter intrinsic neuronal properties (Marder, 2012) by changes in gene expression, the molecular mechanisms through which this is achieved and maintained over developmental timescales needs further understanding.
Ca2+ signals generated by neuronal activity can determine neurotransmitter specification (Spitzer, 2012), synaptic plasticity (O’Hare et al., 2022; Takechi et al., 1998), patterns of neurite growth (Gu and Spitzer, 1995), and gene expression programs (Ciceri et al., 2022; Rosenberg and Spitzer, 2011) over developmental timescales (Arjun McKinney et al., 2022) where output specificity is defined by signal dynamics. Neuromodulators generate intracellular Ca2+ signals by stimulation of cognate G-protein-coupled receptors (GPCRs) linked to the inositol 1,4,5-trisphosphate (IP3) and Ca2+ signalling pathway. IP3 transduces intracellular Ca2+ release through the endoplasmic reticulum (ER) localised ligand-gated ion channel, the inositol 1,4,5-trisphosphate receptor (IP3R; Streb et al., 1984), followed by store-operated Ca2+ entry (SOCE) through the plasma membrane localised Orai channel (Prakriya and Lewis, 2015; Thillaiappan et al., 2019). Cellular and physiological consequences of Ca2+ signals generated through SOCE exhibit different timescales and subcellular localisation from activity-induced signals, suggesting that they alter neuronal properties through novel mechanisms.
Expression studies, genetics, and physiological analysis support a role for IP3/Ca2+ and SOCE in neuronal development and the regulation of adult neuronal physiology across organisms (Hasan and Sharma, 2020; Mitra and Hasan, 2022; Somasundaram et al., 2014). In Drosophila, neuromodulatory signals of neurotransmitters and neuropeptides stimulate cognate GPCRs on specific neurons to generate IP3 followed by intracellular Ca2+ release and SOCE (Agrawal et al., 2013; Megha and Hasan, 2017; Shakiryanova et al., 2011). Genetic and cellular studies identified neuromodulatory inputs and SOCE as an essential component for maturation of neural circuits for flight (Pathak et al., 2015; Venkiteswaran and Hasan, 2009). A focus of this flight deficit lies in a group of central dopaminergic neurons (DANs) that include the PPL1 and PPM3 DANs (Liu et al., 2012) marked by THD’ GAL4, alternately referred to henceforth as flight-promoting DANs (fpDANs). Among the fpDANs, some PPL1 DANs project to the γ2α’1 lobe of the mushroom body (MB; Mao and Davis, 2009), a dense region of neuropil in the insect central brain, that forms a relay centre for flight (Sharma and Hasan, 2020). Axonal projections from Kenyon cells (KCs) carry sensory inputs (Cervantes-Sandoval et al., 2017; Tsao et al., 2018; Yagi et al., 2016) and those from DANs carry internal state information (Mao and Davis, 2009; Riemensperger et al., 2005; Zolin et al., 2021) to functional compartments of the MB, where dopamine release modulates the KC and MB output neuron synapse (MBONs; Aso et al., 2014). The KC-DAN-MBON tripartite synapse carries a dynamically updating representation of the motivational and behavioural state of the animal (Aso and Rubin, 2016; Berry et al., 2015; Owald et al., 2015; Waddell, 2016). Here, we have investigated the molecular mechanisms that underlie the ability of neuromodulatory acetylcholine signals, acting through SOCE during circuit maturation, to determine fpDAN function required for Drosophila flight.
Results
A spatio-temporal requirement for SOCE determines flight
To understand how neuromodulator-stimulated SOCE alters neuronal properties over developmental timescales, we began by refining further the existing spatio-temporal coordinates of SOCE requirement for flight. fpDANs with a requirement for SOCE have been identified earlier by expression of a dominant negative Orai transgene, which renders the channel Ca2+ impermeable, thus abrogating all SOCE as observed in primary neuronal cultures (OraiE180A; Figure 1A; Pathak et al., 2015; Yeromin et al., 2006). These include a smaller subset of THD’GAL4 marked DANs, where acetylcholine promotes dopamine release through the muscarinic acetylcholine receptor (mAchR) by stimulating IP3/Ca2+ signalling (Sharma and Hasan, 2020). OraiE180A expression in a subset of 21–23 DANs marked by THD’ GAL4 (Figure 1B) led to complete loss of flight (Figure 1C) while overexpression of the wildtype Orai transgene had a relatively minor effect (Figure 1—figure supplement 1).
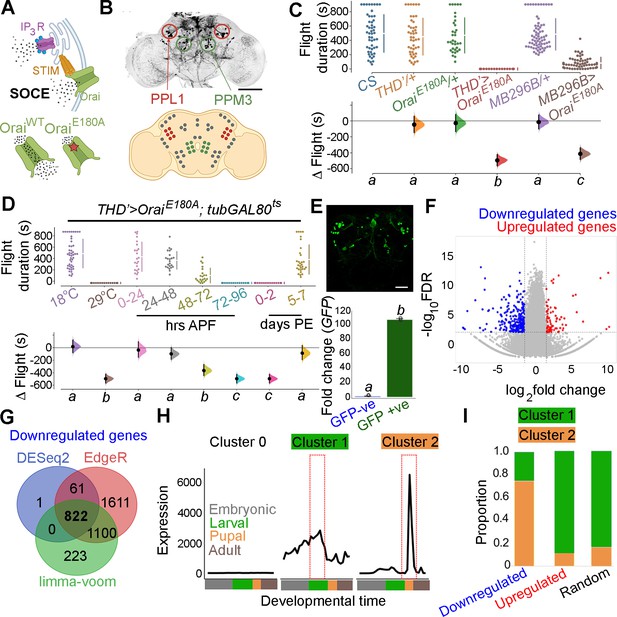
Orai-mediated Ca2+ entry sets the gene expression profile of flight-promoting dopaminergic neurons (DANs) in late development and early adulthood.
(A) A schematic of Ca2+ release through the inositol 1,4,5-trisphosphate receptor (IP3R) and store-operated Ca2+ entry (SOCE) through STIM/Orai (upper panel), followed by representation of the wildtype (Ca2+ permeable) and mutant (Ca2+ impermeable) Orai channels (lower panel). (B) Anatomical location of THD’ DANs in the fly central brain immunolabelled for mCD8GFP (upper panel), followed by a cartoon of central brain DAN clusters. Scale bar indicates 20 μm. PPL1 and PPM3 clusters are labelled in red and green, respectively. (C) Measurement of flight bout durations demonstrates a requirement for Orai-mediated Ca2+ entry in THD’ DANs and in two pairs of PPL1 DANs marked by the MB296B driver. (D) THD’ DANs require Ca2+ entry through Orai at 72–96 hr after puparium formation (APF) and 0–2 d post eclosion to promote flight. In (C) and (D), flight bout durations in seconds (s) are represented as a swarm plot where each genotype is represented by a different colour, and each fly as a single data point. The Δ Flight parameter shown below indicates the mean difference for comparisons against the shared Canton S control and is shown as a Cumming estimation plot. Mean differences are plotted as bootstrap sampling distributions. Each 95% confidence interval is indicated by the ends of the vertical error bars. The same letter beneath each distribution refers to statistically indistinguishable groups after performing a Kruskal–Wallis test followed by a post hoc Mann–Whitney U-test (p<0.005). At least 30 flies were tested for each genotype. (E) THD’ DANs were labelled with cytosolic eGFP (10 μm scale), isolated using fluorescence-activated and sorted (FACS), and validated for enrichment of GFP mRNA by qRT-pCR (lower panel). The qRT-pCR results are from four biological replicates, with different letters representing statistically distinguishable groups after performing a two-tailed t-test (p<0.05). (F) RNA-seq comparison of FAC-sorted populations of GFP-labelled THD’ DANs from THD’>GFP and THD’>GFP;OraiE180A pupal dissected central nervous systems (CNSs). The RNA-seq data is represented in the form of a volcano plot of fold change vs FDR. Individual dots represent genes, coloured in red (upregulated) or blue (downregulated) by greater than onefold. (G) Downregulated genes were identified by three different methods of differentially expressed gene (DEG) analysis, quantified, and compared as a Venn diagram. (H) Gene expression trajectories of SOCE-induced DEGs plotted as a function of developmental time (modENCODE Consortium et al., 2010) and clustered into three groups using k-means analysis. (I) The relative proportion of downregulated, upregulated genes, and a random set of genes found in the clusters described in (H) indicates that 75% of downregulated genes exhibit a pupal peak of expression.
-
Figure 1—source data 1
Raw data for flight assays (C, D), IHC quantification (E), and differential gene expression analysis (F, H, I).
- https://cdn.elifesciences.org/articles/88808/elife-88808-fig1-data1-v1.xlsx
THD’ marks DANs in the PPL1, PPL2, and PPM3 clusters of the adult central brain (Figure 1B) of which the PPL1 (10–12) and PPM3 (6–7) cell clusters constitute the fpDANs (Pathak et al., 2015). Among these 16–19 DANs, two pairs of PPL1 DANs projecting to the γ2α’1 lobes of the MB (marked by the MB296B split GAL4 driver and schematised in Figure 1—figure supplement 2; Aso et al., 2014; Aso and Rubin, 2016) were identified as contributing to the flight deficit to a significant extent (Figure 1C, Figure 1—figure supplement 3). The MB296B DANs form part of a central flight relay centre identified earlier as requiring IP3/Ca2+ signalling (Sharma and Hasan, 2020). That the THD’ neurons are required for flight was further confirmed by inhibiting their function. Acute activation of either a temperature-sensitive Dynamin mutant (Shibirets; Kitamoto, 2001), which blocks exocytosis at an elevated temperature, or acute expression of the tetanus toxin light chain fragment (TeTxLC; Sweeney et al., 1995), which cleaves Synaptobrevin, an essential component of the synaptic release machinery in THD’ neurons, led to severe flight defects (Figure 1—figure supplement 4).
Previous work has demonstrated that intracellular Ca2+ release through the IP3R, which precedes SOCE (Figure 1A), is required during late pupal development in THD’-marked DANs for flight (Pathak et al., 2015; Sharma and Hasan, 2020). The precise temporal requirement for SOCE in THD’ neurons was investigated by inducing OraiE180A transgene expression for specific periods of pupal development and in adults with the tubulinGAL80ts-based temperature-sensitive expression system (TARGET – Temporal And Regional Gene Expression Targeting; McGuire et al., 2004). Approximately 100 hr of pupal development were binned into 24 hr windows, wherein SOCE was abrogated by OraiE180A expression, following which normal development and growth were permitted. Flies were assayed for flight 5 d post eclosion. Abrogation of SOCE by expression of the OraiE180A-dominant-negative transgene at 72–96 hr after puparium formation (APF) resulted in complete loss of flight compared to minor flight deficits observed upon expression of OraiE180A during earlier developmental windows (Figure 1D). Abrogation of flight also occurred upon OraiE180A expression during the first 2 d post-eclosion (see ‘Discussion’), whereas abrogation of SOCE 5 d after eclosion as adults resulted in only modest flight deficits (Figure 1D), indicating that SOCE is required for maturation of THD’-marked flight DANs. Earlier work has shown that loss of SOCE by expression of OraiE180A does not alter the number of DANs or affect neurite projection patterns of PPL1 and PPM3 neurons (Pathak et al., 2015). Of these two clusters, PPM3 DANs project to the ellipsoid body in the central complex (Kong et al., 2010), and PPL1 DANs project to the MB and the lateral horn (Mao and Davis, 2009) and aid in the maintenance of extended flight bouts (Sharma and Hasan, 2020).
Loss of SOCE in late pupae leads to a reorganisation of neuronal gene expression
The molecular consequences of loss of SOCE in THD’ neurons were investigated next by undertaking a comprehensive transcriptomic analysis from fluorescence-activated and sorted (FACS) THD’ neurons with or without OraiE180A expression and marked with eGFP (Figure 1E, Figure 1—figure supplement 5). THD’ neurons were obtained from pupae at 72 ± 6 hr APF (essential SOCE requirement for flight; Figure 1E). Expression analysis of RNA-seq libraries generated from THD’ neurons revealed a reorganisation of the transcriptome upon loss of SOCE (Figure 1F). Expression of 822 genes was downregulated (with a fold change cut-off of < –1) as assessed using three different methods of differentially expressed genes (DEG) analysis (Figure 1G), whereas 137 genes were upregulated (Figure 1—figure supplement 6). To understand whether loss of SOCE affects genes expressed in neurons from the pupal stage, we used the modEncode dataset (Celniker et al., 2009; modENCODE Consortium et al., 2010) to reconstruct developmental trajectories of all genes expressed in THD’ neurons (Figure 1—figure supplement 7). Using an unsupervised clustering algorithm, we classified genes expressed in THD’ neurons into three clusters, where ‘cluster 0’ is low expression throughout development, ‘cluster 1’ exhibits a larval peak in expression, and ‘cluster 2’ exhibits a pupal peak in expression (Figure 1H). The majority of expressed genes (>95%) classify as low expression throughout (cluster 0) and were removed from further analysis. Genes belonging to either the downregulated gene set or the upregulated gene set in SOCE-deficient THD’ neurons and a random set of genes were analysed further. A comparison of the proportion of DEGs classified in the larval and pupal clusters revealed that >75% of downregulated genes belonged to the pupal peak (cluster 2), whereas <20% of either the upregulated genes or a random set of genes classified as belonging to the larval peak (Figure 1I). Moreover, downregulated genes exhibit higher baseline expression in wildtype pupal THD’ neurons (Figure 1—figure supplements 8 and 9). These analyses suggest that SOCE induces expression of a set of genes in THD’ neurons during the late pupal phase, which are subsequently required for flight. These are henceforth referred to as SOCE-responsive genes.
SOCE regulates gene expression through a balance of histone 3 lysine 36 trimethylation and histone 3 lysine 27 trimethylation
Gene Ontology (GO) analysis of SOCE-responsive genes revealed ‘Transcription’, ‘Ion transporters’, ‘Ca2+ dependent exocytosis’, ‘GPCRs’, ‘Synaptic components’, and ‘Kinases’ as top GO categories (Figure 2A). Among these, ‘Transcription’ and ‘Ion transporters’ represented categories with the highest enrichment. Further analysis of genes that classified under ‘Transcription’ revealed SET domain-containing genes that encode histone lysine methyltransferases implicated in chromatin regulation and gene expression (Dillon et al., 2005), and among the SET domain-containing genes, Set1 and Set2 showed distinct downregulation (Figure 2B). One of these genes, the H3K36me3 methyltransferase Set2 (Figure 2C), was identified in a previous RNA-seq from larval neurons with loss of IP3R-mediated Ca2+ signalling (Mitra et al., 2020). RT-PCRs from sorted THD’ neurons confirmed that Set2 levels are indeed downregulated (by 76%) in THD’ neurons expressing OraiE180A and to the same extent as seen upon expression of Set2RNAi (79% downregulation; Figure 2C). Set1 and Set2 perform methyltransferase activity at H3K4 and H3K36 histone residues, respectively (Ardehali et al., 2011; Stabell et al., 2007). Both these markers are epigenetic signatures for transcriptional activation, where H3K4me3 is enriched at the 5′ end of the gene body and H3K36me3 is enriched in the gene bodies of actively transcribed genes; schematised in Figure 2—figure supplement 1. In the adult central nervous system (CNS), Set2, is expressed at fourfold higher levels compared to Set1 (Figure 2—figure supplement 2; modENCODE Consortium et al., 2010). Set2 also shows a steep 5.5-fold increase in expression levels during the pupal to adult transition compared to a 2-fold increase in Set1 (Figure 2—figure supplement 2). These observations, coupled with the previous report of Set2 function downstream of IP3R/Ca2+ signalling in larval glutamatergic neurons (Mitra et al., 2020), led us to pursue the role of Set2 in THD’ neurons.
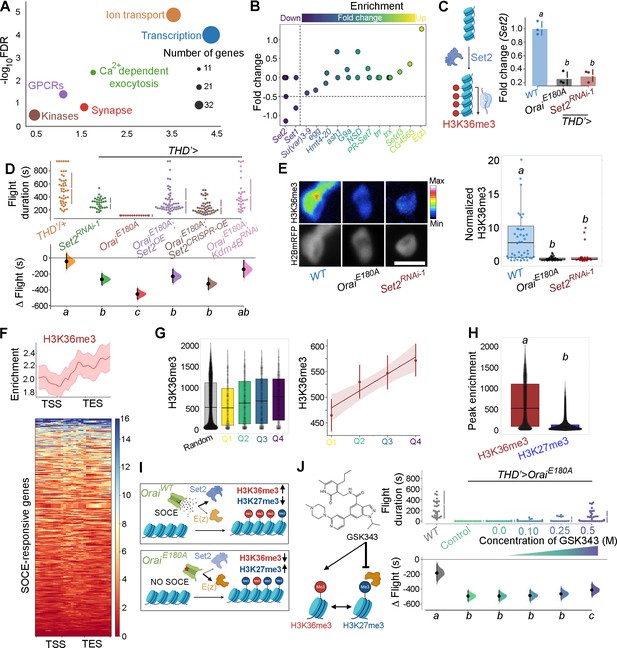
Ca2+ entry through Orai regulates gene expression by Set2-mediated histone modification.
(A) Scatter plot of Gene Ontology (GO) categories enriched in store-operated Ca2+ entry (SOCE)-responsive genes. Individual GO terms are represented as differently coloured circles, with radius size indicating number of genes enriched in that category. (B) Fold change of SET domain containing genes as indicated. Individual circles on the Y-axis for each gene represent transcript variants pertaining to that gene. (C) Transcripts of the H3K36 methyltransferase (left) are significantly diminished (right) in THD’ dopaminergic neurons (DANs) either upon loss of Orai function (THD’>OraiEE180A) or by knockdown of Set2 (THD’>Set2 RNAi). qRT-PCRs were performed from FAC-sorted THD’ DANs with three biological replicates. Individual 2-ΔΔCT values are shown as points. Letters represent statistically distinguishable groups after performing an ANOVA and post hoc Tukey test (p<0.05). (D) Significant rescue of flight bout durations seen in THD’>OraiEE180A flies by overexpression of Set2 and by knockdown of the Kdm4B demethylase indicating a net requirement for H3K36me3. Flight durations of single flies are depicted as swarm plots and the Δ flight parameter is shown below. Both were measured as described in the legend to Figure 1. N = 30 or more flies for each genotype. Letters represent statistically distinguishable groups after performing an ANOVA and post hoc Tukey test (p<0.005). (E) Representative images (upper panel) and quantification (lower panel) from immunostaining of H3K36me3 and H2BmRFP in nuclei of THD’ DANs from at least 10 brains. Scale bar represents 5 μm. The boxplot represents individual H3K36me3/H2BmRFP ratios from each THD’ DAN for each genotype. Letters represent statistically distinguishable groups after performing an ANOVA and post hoc Tukey test (p<0.05). (F) H3K36me3 enrichment over the gene bodies of SOCE-responsive genes in wildtype fly heads represented in the form of a tag density plot. (G) H3K36me3 signal is enriched on WT SOCE-responsive genes with greater downregulation upon loss of Orai function. Individual data points represent WT H3K36me3 ChIP-seq signals from adult fly heads represented as a boxplot (left) and a regression plot (right; Pearson’s correlation coefficient = 0.11), indicating a correlation between extent of downregulation upon loss of SOCE and greater enrichment of H3K36me3. (H) SOCE-responsive genes are enriched in H3K36me3 signal compared to H3K27me3 signal as measured from relevant ChIP-seq datasets. Adult fly head ChIP-seq datasets for measurements in (F–H) were obtained from modEncode (modENCODE Consortium et al., 2010). (I) Schematic representation of how Orai-mediated Ca2+ entry regulates a balance of two opposing epigenetic signatures in developing DANs. (J) Pharmacological inhibition of H3K27me3 using GSKS343 (left) in THD’>OraiEE180A flies results in a dose-dependent rescue of flight bout durations (right). Flight assay measurements from N > 30 flies, are represented as described earlier. Letters represent statistically distinguishable groups after performing an ANOVA and post hoc Tukey test (p<0.005).
-
Figure 2—source data 1
Raw data for GO Analysis (A), Transcript quantification (B), RT-qPCRs (C), flight assays (D, J), IHC quantification (E), and ChIP-Seq peak quantification (F, G, H).
- https://cdn.elifesciences.org/articles/88808/elife-88808-fig2-data1-v1.xlsx
Set2 encodes the only Drosophila methyltransferase which is specific for H3K36 trimethylation (Stabell et al., 2007). To understand the functional significance, if any, of downregulation of Set2 in THD’ neurons upon loss of SOCE, four independent Set2 RNAi constructs were obtained. Knockdown of Set2 in THD’ neurons with all four RNAi lines tested resulted in significant flight defects (Figure 2D, Figure 2—figure supplement 3). Set2 function for flight was additionally verified in the PPL1 DANs projecting to the γ2α’1 lobe of the MB (MB296BGAL4; Figure 2—figure supplement 4). To test the hypothesis that Set2 expression and function for flight is regulated by SOCE, we overexpressed Set2 in the OraiE180A background using GAL4-UAS-driven heterologous Set2WT expression or CRISPR-dCas9::VPR-driven overexpression (Gilbert et al., 2013) from the endogenous locus and measured flight (Figure 2D, data in purple and brown). Flies expressing OraiE180A in the THD’ neurons, which are flightless, exhibit significant rescue in flight bout durations upon Set2 overexpression, through either method (Figure 2D, compare red with purple and brown data), but not upon expression of a control transgene, GCamP6m (Figure 2—figure supplement 5, compare data in green and red). Although the Set2 overexpression rescues were induced all through development, because Orai function drives critical gene expression during 72–96 hr APF (Figure 1D), we concluded that Set2-mediated rescue is also likely to occur during this time window. These data taken together with downregulation of Set2 upon expression of OraiE180A (Figure 2C) support the hypothesis that SOCE-driven expression of Set2 in fpDANs is required for flight. That Set2 function downstream of SOCE occurs specifically through its methyltransferase activity was supported through experiments where knockdown of the demethylase Kdm4B, the primary histone demethylase expressed in these neurons (Figure 2—figure supplement 6), rescued flight in THD’>OraiEE180A flies (Figure 2D, data in pink). Knockdown of the lower expressed Drosophila H3K36 demethylase isoform, Kdm4A, did not show a similar rescue (Figure 2—figure supplement 7, data in pink). Moreover, both Set2 depletion and loss of SOCE (THD’>OraiEE180A) lead to a decrease in the normalised H3K36me3 signal in THD’ neurons (Figure 2E).
To understand whether deficient H3K36me3 is found in the SOCE-responsive genes, we quantified the enrichment of H3K36me3 at SOCE-responsive loci from an H3K36me3 ChIP-seq dataset (modENCODE Consortium et al., 2010) from wildtype adult heads (Figure 2F). We observed an enrichment of the H3K36me3 signal over the gene bodies of the SOCE-responsive genes (Figure 2F). Additionally, genes that were more affected by loss of SOCE (greater extent of downregulation) had a correspondingly higher H3K36me3 signal (Figure 2G) compared with a random set of genes (Figure 2G, data in gray), further indicating that one of the ways SOCE regulates gene expression is through Set2-mediated deposition of H3K36me3.
The gene E(z) that encodes a component of the PRC2 complex (EZH2) which deposits H3K27me3 is upregulated upon loss of SOCE (Figure 2B), suggesting that SOCE could affect additional histone modifications. While H3K36me3 is a marker for transcriptional activation (Krogan et al., 2003), H3K27me3 is a repressive signature which silences transcription (Cai et al., 2021). These two marks are antagonistic as deduced from studies which have shown that deposition of H3K36me3 allosterically inhibits the Polycomb Repressive Complex (PRC2), thereby preventing it from depositing the H3K27me3 signature at the same genomic loci (Finogenova et al., 2020). A comparison of H3K36me3 and H3K27me3 peaks over the 822 SOCE-responsive genes revealed a higher enrichment of H3K36me3 versus H3K27me3 in wildtype fly heads (Figure 2H; modENCODE Consortium et al., 2010), suggesting that robust expression of these genes in adult neurons occurs through an SOCE-dependent mechanism initiated in late pupae (schematised in Figure 2I). To test this hypothesis, we fed SOCE-deficient flies (THD’GAL4>OraiEE180A) GSK343, a pharmacological inhibitor (Verma et al., 2012) of the EZH2 component of PRC2 that is known to reduce H3K27me3 chromatin marks and performed flight assays. Feeding of GSK343 lead to partial rescue of flight in THD’>OraiEE180A flies in a dose-dependent manner with maximal flight bout durations of up to 400 s (Figure 2J, Figure 2—figure supplement 8).
Normal cellular responses of PPL1 DANs require Orai-mediated SOCE and H3K36 trimethylation
Next we investigated Ca2+ responses in SOCE and H3K36 trimethylation-deficient THD’ PPL1 DANs upon stimulation with a neuromodulatory signal for the mAChR. It is known that THD’-marked PPL1 DANs receive cholinergic inputs that stimulate store Ca2+ release through the IP3R, leading to SOCE through Orai (Figure 3A; Ebihara et al., 2006; Sharma and Hasan, 2020). In support of this idea, either expression of OraiE180A or knockdown of the IP3R attenuated the composite ER-Ca2+ release plus SOCE response to carbachol (CCh), a mAChR agonist, in PPL1 DANs (Figure 3B–D, Figure 3—figure supplement 1). Ex vivo brain preparations used here for in situ Ca2+ imaging of fpDANs are not viable in the absence of extracellular Ca2+. Hence, measurement of store-Ca2+ release and SOCE independent of each other was not possible. Importantly, knockdown of Set2 also abrogated the Ca2+ response to CCh (Figure 3B–D), whereas overexpression of a transgene encoding Set2 in THD’ neurons either with loss of SOCE (OraiE180A) or with knockdown of the IP3R (itprRNAi) leads to significant rescue of the Ca2+ response, indicating that Set2 is required downstream of SOCE (Figure 3B–D) and IP3/Ca2+ signalling (Figure 3—figure supplement 1).
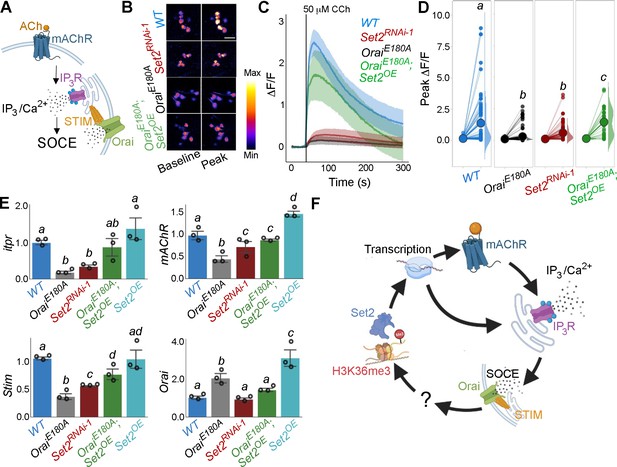
Orai-mediated Ca2+ entry potentiates cellular Ca2+ responses to cholinergic inputs through Set2 and a transcriptional feedback loop.
(A) A schematic of intracellular Ca2+ signalling downstream of neuromodulatory signalling where activation of muscarinic acetylcholine receptor (mAchR) stimulates intracellular Ca2+ release through the inositol 1,4,5-trisphosphate receptor (IP3R) followed by store-operated Ca2+ entry (SOCE) through STIM/Orai. (B) Cholinergic inputs by addition of carbachol (CCh) evoke Ca2+ signals as measured by change in the fluorescence of GCaMP6m in THD’ dopaminergic neurons (DANs) of ex vivo brains. Representative GCaMP6m images of THD’ DANs are shown with baseline and peak-evoked responses in the indicated genotypes. Scale bar = 10 μM. (C) Median GCaMP6m responses plotted as a function of time. A shaded region around the solid line represents the 95% confidence interval from 4 to 5 cells imaged per brain from 10 or more brains per genotype. (D) Individual cellular responses depicted as a paired plot where different letters above indicate statistically distinguishable groups after performing a Kruskal–Wallis test and a Mann–Whitney U-test (p<0.05). (E) qRT-PCR measurements of itpr, mAChR, Stim, and Orai from FAC-sorted THD’ DANs obtained from three biological replicates of appropriate genetic backgrounds. The genotypes include DANs with loss of cellular Ca2+ responses (THD’>OraiEE180A; and THD’>Set2RNAi-1), rescue of cellular Ca2+ response by overexpression of Set2 (THD’>OraiEE180A; Set2OE), and Set2 overexpression. Bar plots indicate mean expression levels in comparison to rp49, with individual data points represented as hollow circles. The letters above indicate statistically indistinguishable groups after performing a Kruskal–Wallis test and a Mann–Whitney U-test (p<0.05). (F) Schematic representation of a transcriptional feedback loop downstream of cholinergic stimulation in THD’ DANs.
-
Figure 3—source data 1
Raw data for imaging quantification (C, D) and RT-qPCRs (E).
- https://cdn.elifesciences.org/articles/88808/elife-88808-fig3-data1-v1.xlsx
An understanding of how Set2 might rescue cellular IP3/Ca2+ signalling was obtained in an earlier study where it was demonstrated that Set2 participates in a transcriptional feedback loop to control the expression of key upstream components such as the mAChR and the IP3R in a set of larval glutamatergic neurons (Mitra et al., 2020). To test whether Set2 acts through a similar transcriptional feedback loop in the current context, THD’ DANs were isolated using FACS from appropriate genetic backgrounds and tested for expression of key genes required for IP3/Ca2+ signalling (mAChR and itpr) and SOCE (Stim and Orai). Loss of SOCE upon expression of OraiE180A leads to a significant decrease in the expression of itpr (72% downregulation; Figure 3E) and mAChR (56% downregulation; Figure 3E) in THD’ DANs. As expected, Orai expression (Figure 3E) increased twofold presumably due to overexpression of the OraiE180A transgene. Importantly, knockdown of Set2 in THD’ neurons also led to downregulation of itpr (43%), mAChR (78%), and Stim (61%) (Figure 3E). Overexpression of Set2 in the background of OraiE180A led to a rescue in the levels of itpr (69% increase), mAChR (43% increase), and Stim (40% increase) (Figure 3E). Overexpression of Set2 in wildtype THD’ neurons resulted in upregulation of mAChR and Orai (Figure 3E). These findings indicate that while SOCE regulates Set2 expression (Figure 2C), Set2 in turn functions in a feedback loop to regulate expression of key components of IP3/Ca2+ (mAchR and IP3R) and SOCE (STIM and Orai) (Figure 3E). Thus, ectopic Set2 overexpression in SOCE-deficient THD’ neurons leads to increase in expression of key genes that facilitate intracellular Ca2+ signalling and SOCE (schematised in Figure 3F). Direct measures of Orai-channel function under conditions of altered Set2 expression are needed in future to assess how the feedback loop alters CCh-induced ER-Ca2+ release and SOCE independent of each other (see ‘Limitations of this study’). While we assume that the observed changes in gene expression translate to alterations in protein levels, direct measurement of protein levels specifically from THD’ neurons needs to be addressed in the future.
Trithorax-like (Trl) is an SOCE-responsive transcription factor in THD’ DANs
The data so far identify SOCE as a key developmental regulator of the neuronal transcriptome in THD’-marked DANs, where it upregulates Set2 expression and thus enhances the activation mark of H3K36 trimethylation on specific chromatin regions. However, the mechanism by which SOCE regulates expression of Set2 and other relevant effector genes remains unresolved (Figure 3F). In mammalian T cells, SOCE leads to de-phosphorylation of the NFAT (nuclear factor of activated T cells) family of transcription factors (TFs) by Ca2+/calmodulin-sensitive phosphatase calcineurin, followed by their nuclear translocation and ultimately transcription of relevant target genes (Hogan et al., 2003). Unlike mammals, the Drosophila genome encodes a single member of the NFAT gene family, which does not possess calcineurin binding sites, and is therefore insensitive to intracellular Ca2+ and SOCE (Keyser et al., 2007).
In order to identify TFs that regulate SOCE-mediated gene expression in Drosophila neurons, we examined the upstream regions (up to 10 kb) of SOCE-regulated genes using motif enrichment analysis (Zambelli et al., 2009) for TF binding sites (Figure 4A). This analysis helped identify several putative TFs with enriched binding sites in the regulatory regions of SOCE-regulated genes (Figure 4B, Figure 4—figure supplement 1) which were then analysed for developmental expression trajectories (Hu et al., 2017) in the Drosophila CNS (Figure 4B, lower panel). Among the top 10 identified TFs, the homeobox TF Trl/GAF had the highest enrichment value, lowest p-value (Figure 4B), and was consistently expressed in the CNS through all developmental stages, with a distinct peak during pupal development (Figure 4B, lower panel). To test the functional significance of Trl/GAF, we tested flight in flies with THD’-specific knockdown of Trl using two independent RNAi lines (Figure 4C) as well as in existing Trl mutant combinations that were viable as adults (Figure 4—figure supplement 2). While homozygous trl null alleles are lethal, trans-heterozygotes of two different hypomorphic alleles were viable and exhibit significant flight deficits (Figure 4C, Figure 4—figure supplement 2). Moreover, the flight deficits caused by TrlRNAi specifically in the THD’ neurons could be rescued by Set2 overexpression (Figure 4C, compare data in red and brown).

Identification of trithorax-like (Trl) as a store-operated Ca2+ entry (SOCE)-responsive transcription factor (TF).
(A) Schematic of motif enrichment analysis for identification of putative SOCE-dependent TFs. (B) Candidate TFs identified (upper panel) and their expression through development (lower panel; modEncode; modENCODE Consortium et al., 2010) suggests Trl as a top SOCE-responsive candidate TF. (C) Genetic depletion of Trl in THD’ dopaminergic neurons (DANs) results in significant flight defects, which can be rescued by overexpression of Set2. (D) Heteroallelic combination of the Trl hypomorphic allele (trl13C) with a STIM deficiency causes significant flight deficits, which can be rescued by overexpression of STIM or Set2. (E) qRT-PCRs measured relative to rp49 show reduced Set2, itpr, and mAChR levels in THD’ DANs upon Trl knockdown with TrlRNAi-1. Individual data points of four biological replicates are shown as circles and mean expression level as a bar plot (± SEM). The letters above indicate statistically indistinguishable groups after performing a Kruskal–Wallis test and a Mann–Whitney U-test (p<0.05). (F) A representative western blot (left) showing reduced H3K36me3 levels in a Trl mutant combination. Quantification of H3K36me3 from three biological replicates of WT (control) and Trl mutant brain lysates (panel on the right). Letters indicate statistically indistinguishable groups after performing a two-tailed t-test (p<0.05). (G) Knockdown of Trl in THD’ neurons attenuates Ca2+ response to carbachol (CCh) as shown in representative images of GCamP6m fluorescence quantified in (H, I). Scale bar = 10 μM. Set2 overexpression in the background of THD’>TrlRNAi rescues the cholinergic response (G–I). Quantification of Ca2+ responses is from 10 or more brains per genotype and performed as described in the legend to Figure 3. The letters above indicate statistically indistinguishable groups after performing a Kruskal–Wallis test and a Mann–Whitney U-test (p<0.05).
-
Figure 4—source data 1
Raw data for TF motif enrichment analysis (B), flight assays (C, D), RT-qPCRs (E), western blot quantifications (F),and imaging quantifications (H, I).
- https://cdn.elifesciences.org/articles/88808/elife-88808-fig4-data1-v1.xlsx
To further test Trl function, as an effector of intracellular Ca2+ signalling and SOCE, we investigated genetic interactions of trl13C, a hypomorphic mutant-recessive Trl allele, with an existing deficiency for the ER-Ca2+ sensor STIM (STIMKO), an essential activator of the Orai channel (Wang et al., 2010) as well as existing mutants for an intracellular ER-Ca2+ release channel, the IP3R (Joshi et al., 2004). While STIMKO/+ flies demonstrate normal flight bout durations, adding a single copy of the trl13C allele to the STIMKO heterozygotes (STIMKO/+; trl13C/+ trans-heterozygotes) resulted in significant flight deficits, which could be rescued by overexpression of either STIM or Set2 (Figure 4D). The rescue of STIMKO/+; trl13C/+ with STIMOE (Figure 4D) indicates that SOCE, driven by STIMOE, activates residual Trl encoded by a copy of Trl+ in this genetic background (trl13c/+) to rescue flight. The role of Trl as an SOCE-regulated TF is further supported by rescue of flight in STIMKO/+; trl13c/+ flies by overexpression of Set2 (Figure 4D). Set2 expression in THD’ neurons was demonstrated earlier as requiring Orai-mediated Ca2+ entry (Figure 2B and C). These genetic data are consistent with the positive feedback loop proposed earlier (Figure 3F). We hypothesise that Trl is non-functional upon expression of OraiE180A due to reduced SOCE. Loss of Trl function in turn downregulates Set2 expression. A single copy of trl13C placed in combination with a single copy of various itpr mutant alleles also showed a significant reduction in the duration of flight bouts (Figure 4—figure supplement 3), further supporting a role for intracellular Ca2+ signalling in Trl function. Flight deficits observed upon specific expression of TrlRNAi in THD’ neurons (Figure 4C) indicate the direct requirement of Trl in fpDANs. Taken together, these findings provide good genetic evidence for Trl as an SOCE-responsive TF in THD’ neurons. Due to the strong flight deficit observed by expression of OraiE180A, we were unable to test genetic interactions of Trl with Orai directly. OraiRNAi strains exhibit off-target effects and Orai hypomorphs are unavailable.
The requirement of Trl for SOCE-dependent gene expression was tested directly by measuring Set2 transcripts in FACS-sorted THD’ neurons with knockdown of Trl (THD’>TrlRNAi). Set2 has 20 Trl binding sites in a 2 kb region upstream of the transcription start site (Figure 4—figure supplement 4) and knockdown of Trl resulted in downregulation of Set2 (32%) and the Set2-regulated genes itpr (56%) and mAchR (73%; Figure 4E). Moreover, a trans-heterozygotic combination of hypomorphic Trl alleles (trl13C/62) had markedly reduced levels of brain H3K36me3 (Figure 4F).
To test whether Trl drives cellular function in the fpDANs, we stimulated the mAChR with CCh and measured Ca2+ responses in fpDANs with knockdown of Trl. Compared to WT fpDANs, which respond robustly to cholinergic stimulation, TrlRNAi-expressing DANs exhibit strongly attenuated responses (Figure 4G–I). Overexpression of Set2 in the TrlRNAi background rescued the cholinergic response (Figure 4G–I). The rescue of both flight (Figure 4C and D) and the cholinergic response (Figure 4G–I) by overexpression of Set2 in flies with knockdown of Trl in THD’ neurons (THD’>TrlRNAi) confirms that Trl acts upstream of Set2 to ensure optimal neuronal function and flight.
The results obtained so far indicate that Trl functions as an intermediary TF between SOCE and its downstream effector gene Set2. Overexpression of Trl, however, is unable to rescue the loss of flight caused by loss of SOCE (THD’>OraiEE180A; Figure 5A). Moreover, Trl transcript levels are unchanged in the OraiE180A condition (Figure 5B). These data could either mean that the phenotypes observed upon loss of Trl are independent of Orai-Ca2+ entry or that Trl requires Ca2+ influx through SOCE for its function to go from an ‘inactive’ form to an ‘active’ form (schematised in Figure 5C). We favour the latter interpretation because the key transcripts downregulated by expression of OraiE180A (Set2, itpr, and mAchR) were also downregulated upon knockdown of Trl in THD’ neurons (see Figures 3E and 4E).
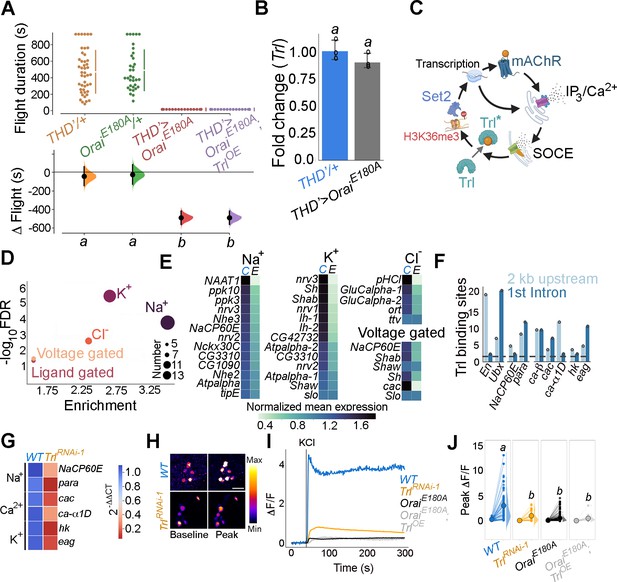
Trithorax-like (Trl) activity downstream of store-operated Ca2+ entry (SOCE) is required for THD’ dopaminergic neuron (DAN) activity.
(A) Overexpression of WT Trl is insufficient to rescue flight deficits in THD’>OraiEE180A flies as evident from flight bout measurements in the indicated genotypes. Flight assay measurements are as described earlier. (B) Trl transcript levels are not altered in THD’>OraiEE180A neurons with loss of SOCE. qRT-PCR data are measured relative to rp49. The bar plot indicates mean expression levels, with individual data points represented as circles. The letters above indicate statistically indistinguishable groups from three independent biological replicates after performing a two-tailed t-test (p<0.05). (C) Schematic with possible Ca2+- mediated activation of Trl downstream of Orai-mediated Ca2+ entry (SOCE). (D). Genes encoding ion channels are enriched among SOCE-responsive genes in THD’ DANs as determined by Gene Ontology (GO) analysis. Circles of varying radii are scaled according to the number of genes enriched in that category. (E) Downregulation of individual ion channel genes depicted as a heatmap. (F) Number of Trl binding sites (GAGA repeats) shown as a bar plot in the regulatory regions (2 kb upstream or first intron) of key SOCE-responsive ion channel genes compared to known Trl targets (En and Ubx). The dashed line indicates the average expected number of Trl targets. (G) Heatmap of 2^-ΔΔCT values measured using qRT-PCRs from sorted THD’ DANs with knockdown of Trl. (H) Representative images of KCl-induced depolarising responses in THD’ DANs with knockdown of Trl. (scale bar = 10 μM) quantified in (I, J). Quantification of Ca2+ responses is from 10 or more brains per genotype and performed as described in the legend to Figure 3. The letters above indicate statistically indistinguishable groups after performing a Kruskal–Wallis test and a Mann–Whitney U-test (p<0.05).
-
Figure 5—source data 1
Raw data for flight assays (A), RT-qPCRs (B), GO analysis (D), transcript quantfication from RNA-Seq (E, G), TF bindiing site analysis (F),and imaging quantifications (I, J).
- https://cdn.elifesciences.org/articles/88808/elife-88808-fig5-data1-v1.xlsx
To understand how Orai-mediated Ca2+ entry might activate Trl, we went back to previously known biochemical characterisation of Trl. Although Trl does not possess a defined Ca2+ binding domain, it interacts with a diverse set of proteins, primarily through its BTB-POZ domain (Figure 5—figure supplement 1) as demonstrated earlier by affinity purification of Trl from embryonic extracts followed by high-throughput mass spectrometry (Lomaev et al., 2017). Among the identified interacting partners, we focused on kinases, keeping in mind an earlier study in Drosophila indicating phosphorylation of Trl at a threonine residue (T237; Zhai et al., 2008). Analysis of the Trl interactome revealed several kinases, which were expressed to varying extents in the THD’ neurons (Figure 5—figure supplement 2), including the Ca2+-dependent CamKII, implicated earlier in flight in THD’ neurons (Ravi et al., 2018). Loss of CamKII in THD’ neurons by RNAi-mediated knockdown or through expression of a peptide inhibitor (Ala; Mehren and Griffith, 2004) resulted in significant flight deficits (Figure 5—figure supplement 3). To test whether CamKII activation downstream of SOCE is required for flight, we expressed a constitutively active version of CamKII (T287D; Kadas et al., 2012) in the background of THD’>OraiEE180A. The phosphomimetic CamKIIT287D point mutation renders CamKII activity Ca2+ independent (Malik and Hodge, 2014). Acute expression of CamKIIT287D in the OraiE180A background using the TARGET system (McGuire et al., 2004) resulted in a weak rescue of flight when implemented from 72 to 96 hr APF (Figure 5—figure supplement 4), whereas overexpression of WT CamKII or a phosphorylation-incompetent allele (CamKIIT287A) failed to rescue flight (Figure 5—figure supplement 4). These data support a model (schematised in Figure 5C) wherein Ca2+ influx through SOCE sustains Trl activation, in part through CamKII, leading to expression of Set2 followed by increased levels of chromatin H3K36me3 marks that drive further downstream gene expression changes through a transcriptional feedback loop. Our data do not rule out activation of other Ca2+-sensitive mechanisms for activation of Trl. Moreover, rescue of flight in Trl mutant/knockdown conditions by STIM overexpression (Figure 4C and D) suggests the presence of additional SOCE-responsive TFs in fpDANs.
Trl function downstream of SOCE targets neuronal activity through changes in ion channel gene expression including VGCCs
Next we investigated the extent to which the identified SOCE-Trl-Set2 mechanism impacts fpDAN function. ‘Ion transport’ is among the top GO terms identified in SOCE-responsive genes (Figure 2A). Indeed, multiple classes of ion channel genes, including several Na+, K+, and Cl- channels, are downregulated in fpDANs upon expression of OraiE180A (Figure 5D and E). Regulation of ion channel gene expression by Trl was indicated from the enrichment of Trl binding sites in regulatory regions of some ion channel loci (Figure 5F). We purified fpDANs with knockdown of Trl (THD’>TrlRNAiRNAi-1) and measured expression of a few key voltage-gated ion channel genes (Figure 5F). NaCP60E, para (Na+ channels), cac, ca-α1D (VGCC subunits), hk, and eag (outward-rectifying K+ channels) are all downregulated between 0.6- and 0.9-fold upon Trl knockdown (Figure 5G) as well as upon loss of SOCE (Figure 5B). Taken together, these results indicate that the expression of key voltage-gated ion channel genes which are required for the depolarisation-mediated response, maintenance of electrical excitability, and neurotransmitter release is a focus of the transcriptional program set in place by SOCE-Trl-Set2.
To test the functional consequences of altered ion channel gene expression downstream of Trl, neuronal activity of the fpDANs was tested by means of KCl-evoked depolarisation. PPL1 DANs exhibited a robust Ca2+ response that was lost upon knockdown of Trl (Figure 5H–J, data in orange). In consonance with the earlier behavioural experiments (Figure 5A), overexpression of Trl+ in THD+ DANs did not rescue the KCl response in PPL1 DANs with loss of SOCE (OraiE180A; TrlOE, Figure 5I and J), further reinforcing the hypothesis that Trl activity in fpDANs is dependent on Ca2+ entry through Orai.
Response to KCl is also lost in PPL1 neurons by knockdown of Set2 and restored to a significant extent by overexpression of Set2 in THD’ DANs lacking SOCE (OraiE180A;Set2OE; Figure 6A–C). We confirmed that the KCl response of PPL1 neurons requires VGCC function either by treatment with nimodopine (Xu and Lipscombe, 2001), an L-type VGCC inhibitor, or by knockdown of a conserved VGCC subunit cac (cacRNAi). Both forms of perturbation abrogated the depolarisation response to KCl in PPL1 neurons (Figure 6D–F, data in purple or orange, respectively). Moreover, the Ca2+ entry upon KCl-mediated depolarisation in PPL1 neurons is a cell-autonomous property as evident by measuring the response after treatment with the Na+ channel inhibitor, tetrodotoxin (TTX; Figure 6D–F, data in magenta).
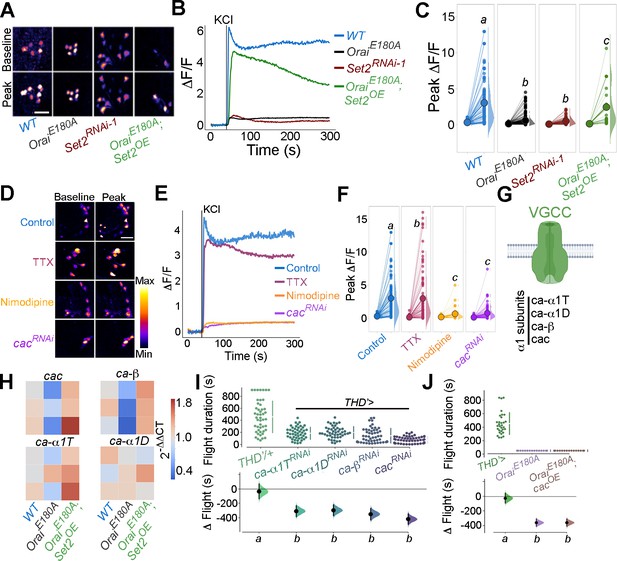
Flight-promoting central dopaminergic neuron (fpDAN) excitability requires Orai-mediated Ca2+ entry acting through Set2-mediated VGCC gene expression.
KCl-induced depolarising responses in fpDANs of the indicated genotypes measured using GCaMP6m indicate a requirement for Orai and Set2. Representative images (A). Scale bar = 10 μM, quantified in (B) and (C). KCl-evoked responses are modulated upon treatment with 10 μM tetrodotoxin (TTX) (magenta), 10 μM nimodipine (orange), and upon cacRNAi (purple), representative images (scale bar = 10 μM) (D), quantified in (E). Median KCl-evoked GCaMP6m responses plotted as a function of time. The solid line indicates the time point of addition of 70 mM KCl. KCl responses are quantified as a paired plot of peak responses and (F) with letters representing statistically indistinguishable groups as measured using a Kruskal–Wallis test and post hoc Mann–Whitney U-test (p<0.05). Quantification of Ca2+ responses is from 10 or more brains per genotype and performed as described in the legend to Figure 3. (G) Schematic of a typical VGCC and its constituent subunits. (H) Heatmap of 2^-ΔΔCT values measured using qRT-PCRs from sorted THD’ DANs shows a reduction in the expression of VGCC subunit genes upon loss of Orai function and a rescue by Set2 overexpression. (I) Flight assays representing the effect of various VGCC subunit gene RNAis showing flight defects to varying extents. Overexpression of the key VGCC subunit gene-cac is required (I) but not sufficient (J) for restoring flight defects caused by loss of Orai function. Flight assay measurements are as described earlier.
-
Figure 6—source data 1
Raw data for imaging quantitations (B, C, E, F), RT-qPCRs (H),and flight assays and (I, J).
- https://cdn.elifesciences.org/articles/88808/elife-88808-fig6-data1-v1.xlsx
Having confirmed that the Ca2+ response towards KCl depolarisation requires VGCCs, we tested the expression level of key components of Drosophila VGCCs (Figure 6G) in THD’ DANs, including cacophony (cac), ca-α1D, ca-α1T, and ca-β. All four subunits of Drosophila VGCC are downregulated upon loss of SOCE (OraiE180A; Figure 6H) in THD’ DANs, whereas overexpression of Set2 in the background of OraiE180A restored expression of the four VGCC subunits tested (Figure 6H), thus explaining recovery of the KCl response upon Set2 overexpression in PPL1 neurons lacking SOCE (OraiE180A; Figure 6A–C).
The functional significance of downregulation of VGCC subunits by the SOCE-Trl-Set2 pathway was tested directly by knockdown of the four VGCC subunits independently in PPL1 neurons followed by measurement of flight bout durations. Significant loss of flight was observed with knockdown of each subunit (Figure 6I) though in no case was the phenotype as strong as what is observed with loss of SOCE (OraiE180A; Figure 1D). These data suggest that in addition to VGCC subunit expression, appropriate expression of other ion channels in the fpDANs is of functional significance. This idea is further supported by the observation that loss of flight upon loss of SOCE cannot be restored by overexpression of cac alone (Figure 6J), indicating that optimal neuronal activity requires an ensemble of genes, including ion channels, whose expression is regulated by SOCE-Trl-Set2.
THD’ DANs require SOCE for developmental maturation of neuronal activity
The functional relevance of changes in neuronal activity during pupal maturation of the PPL1-dependent flight circuit was tested next. Inhibition of THD’ DANs from 72 to 96 hr APF using acute induction of the inward-rectifying K+ channel Kir2.1 (Johns et al., 1999) or optogenetic inhibition through the hyperpolarising Cl- channel GtACR2 (Govorunova et al., 2015) resulted in significant flight deficits (Figure 7A, data in dark or light green). Similar flight defects were recapitulated upon hyperactivation of THD’ neurons through either optogenetic (CsChrimson; Klapoetke et al., 2014) or thermogenetic (TrpA1; Viswanath et al., 2003) stimulation (Figure 7A, data in orange or red), indicating that these neurons require balanced neuronal activity during a critical window in late pupal development, failing which the flight circuit malfunctions. To test whether restoring excitability in fpDANs lacking SOCE (THD’>OraiEE180A) is sufficient to restore flight, we induced hyperexcitability by overexpressing NachBac (Nitabach et al., 2006), a bacterial Na+ channel. NachBac expression rescued both flight (THD’>OraiEE180A; Figure 7B) and excitability (Figure 7C–E). A partial rescue of flight was also obtained by optogenetic stimulation of neuronal activity through activation of THD’>OraiEE180A DANs using CsChrimson either 72–79 hr APF or 0–2 d post eclosion (Figure 7E). In this case, flight rescues were accompanied by a corresponding rescue of CsChrimson activation-induced Ca2+ entry (Figure 7F and G). Moreover, inducing neuronal hyperactivation with indirect methods such as excess K+ supplementation (Figure 7—figure supplement 1) or impeding glial K+ uptake, by genetic depletion of the glial K+ channel sandman (Weiss et al., 2019; Figure 7—figure supplement 2), partially rescued flight in animals lacking SOCE in the THD’ DANs. Together, these results indicate that SOCE, through Trl and Set2 activity, determines activity in fpDAN during circuit maturation by regulating expression of voltage-gated ion channel genes, like cac (see ‘Discussion’).
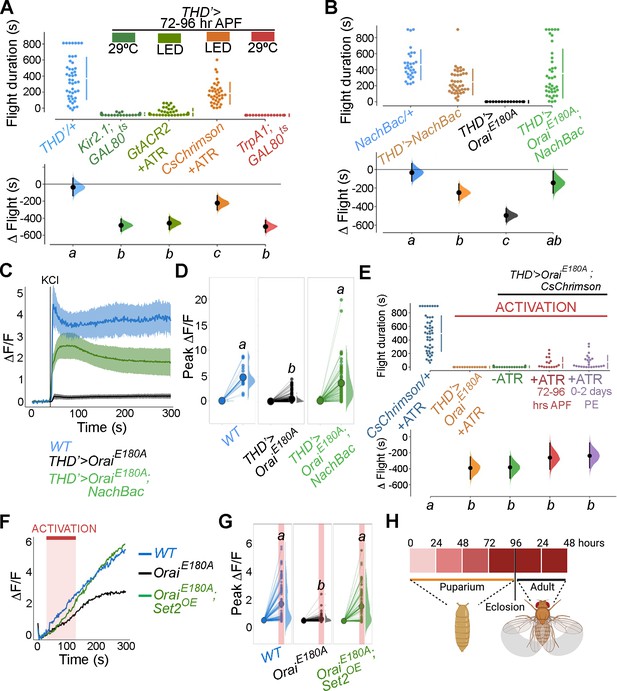
Store-operated Ca2+ entry (SOCE)-mediated gene expression sets the excitability threshold during pupal development.
(A) Altering excitability in THD’ dopaminergic neurons (DANs) using Kir2.1 or GtACR2-mediated inhibition or CsChrimson or TrpA1 during the critical 72–96 hr after puparium formation (APF) developmental window results in significant flight defects. Orai loss-of-function phenotypes can be rescued by overexpression of NachBac or CsChrimson in terms of flight bout durations (B, E) and depolarising KCl responses (C, D, F, G). (H) Schematic of Orai-mediated Ca2+ entry regulating expression of key genes that regulate the excitability threshold of dopaminergic neurons regulating flight during a critical developmental window. Flight assays are represented as described earlier (n > 30). Ca2+ responses were quantified as described in the legend to Figure 3 and were from 10 or more brains per genotype. Letters above each genotype represent statistically indistinguishable groups as measured using a Kruskal–Wallis test and post hoc Tukey test (p<0.05).
-
Figure 7—source data 1
Raw data for flight assays (A, B, E), and imaging quantifications (C, D, F, G).
- https://cdn.elifesciences.org/articles/88808/elife-88808-fig7-data1-v1.xlsx
fpDANs also require a balance between H3K36me3/H3K27me3-mediated epigenetic regulation (Figure 2K). To understand whether this epigenetic balance affects flight through modulation of neuronal excitability, we measured KCl-induced depolarising responses of PPL1 DANs in Orai-deficient animals (THD’>OraiEE180A) fed on the H3K27me3 antagonist GSK343 (0.5 mM). GSK343-fed animals were sorted into two groups (fliers/non-fliers) on the basis of flight bout durations of greater or lesser than 30 s (Figure 7—figure supplement 3) and tested for responses to KCl (Figure 7—figure supplement 3C). THD’>OraiEE180A flies fed on GSK343 showed a rescue in KCl responses, with a clearly enhanced response in the fliers compared to the non-fliers (Figure 7—figure supplement 3C), thereby reiterating the hypothesis that SOCE-mediated regulation of activity in these neurons acts through epigenetic regulation between opposing histone modifications.
THD’ DANs require SOCE-mediated gene expression for axonal arborisation and neuromodulatory dopamine release in the MB γ lobe
Next, we revisited how loss of activity during the critical maturation window of 72–96 hr APF and 0–2 d post-eclosion affects axonal arborisation of fpDANs that innervate the γ lobe. From previous work, we know that major axonal branches of the fpDANs reach the γ lobe normally in THD’>OraiEE180A animals (Figure 8A and B; Pathak et al., 2015). Neuronal activity can drive changes in neurite complexity and axonal arborisation (Depetris-Chauvin et al., 2011) especially during critical developmental periods (Sachse et al., 2007). To understand whether Orai-mediated Ca2+ entry and downstream gene expression through Set2 affects this activity-driven parameter, we investigated the complexity of fpDAN presynaptic terminals within the γ2α’1 lobe MB using super-resolution microscopy (Figure 8A and B). Striking changes in the neurite volume upon expression of OraiE180A were observed. Importantly, these changes could be rescued to a significant extent by restoring either Set2 (OraiE180A; Set2OE) or by inducing hyperactivity through NachBac expression (OraiE180A; NachBacOE; Figure 8C and D).
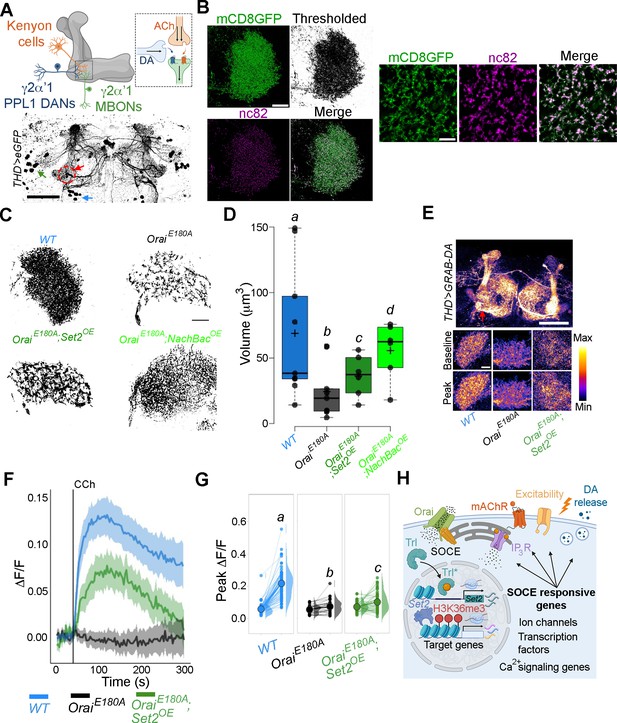
THD’ dopaminergic neurons (DANs) require store-operated Ca2+ entry (SOCE)-mediated gene expression for axonal arborisation and neuromodulatory dopamine release in the mushroom body (MB) γ lobe.
(A) Schematic of a KC-DAN-MBON tripartite synapse at the γ2α’1 MB lobe (upper), innervated by flight-promoting central dopaminergic neurons (fpDANs) (lower). The γ2α’1 MB lobe is marked by a red circle and arrow, and the fpDAN clusters are marked with a green arrow (PPL1) and blue arrow (PPM3). Scale bar = 50 μM. (B) Representative images of the MB γ lobe marked with THD’-driven mCD8GFP (green) and immunolabelled with anti-nc82 (Brp) antibody (magenta) to mark the presynaptic terminals. Scale bar = 5 μm. (C) Representative optical sections through the MB γ lobe from the indicated genotypes. Changes in axonal arborisation observed were quantified as total projection volume in (D, E). Carbachol (CCh)-evoked DA release measured at the γ2α’1 MB lobe using the GRAB-DA sensor expressed in THD’ DANs. Representative GRAB-DA images of THD’ DANs are shown with baseline and peak-evoked responses in the indicated genotypes. Scale bar = 10 μM. (F) Median GRAB-DA responses plotted as a function of time. A shaded region around the solid line represents the 95% confidence interval from 10 or more brains per genotype. (G) Individual cellular responses depicted as a paired plot of peak responses where different letters above indicate statistically distinguishable groups after performing a Kruskal–Wallis test and a Mann–Whitney U-test. (H) Schematic describing how Orai-mediated Ca2+ entry regulates expression of key genes that control neuronal activity in fpDANs.
-
Figure 8—source data 1
Raw data for imaging quantifications (D, F, G).
- https://cdn.elifesciences.org/articles/88808/elife-88808-fig8-data1-v1.xlsx
To understand whether reduced axonal arborisation within the γ lobe has functional consequences, we measured CCh-evoked DA release at the γ2α’1 region of the MB (Figure 8A, red arrow). For this purpose, we used the dopamine sensor GRAB-DA (Sun et al., 2018). Loss of SOCE (THD’>OraiEE180A) attenuated DA release (Figure 8E–G), which could be partially rescued by overexpression of Set2. Taken together these data identify an SOCE-dependent gene regulation mechanism acting through the TF Trl, and the histone modifier Set2 for timely expression of genes that impact diverse aspects of neuronal function, including neuronal activity, axonal arborisation, and sustained neurotransmitter release (schematised in Figure 8H).
Discussion
Over the course of development, neurons define an excitability set point within a dynamic range (Truszkowski and Aizenman, 2015), which stabilises existing connections (Mayseless et al., 2023) and enables circuit maturation (Johnson-Venkatesh et al., 2015). The setting of this threshold for individual classes of neurons is based on the relative expression of various ion channels. In this study, we identify an essential role for the store-operated Ca2+ channel Orai in determining ion channel expression and neuronal activity in a subset of DANs central to an MB circuit for Drosophila flight. Orai-mediated Ca2+ entry, initiated by neuromodulatory acetylcholine signals, is required for amplification of a signalling cascade, which begins by activation of the homeo-box TF Trl, followed by upregulation of several genes, including Set2, a gene that encodes an enzyme for an activating epigenetic mark, H3K36me3. Set2 in turn establishes a transcriptional feedback loop to drive expression of key neuronal signalling genes, including a repertoire of voltage-gated ion channel genes required for neuronal activity in mature PPL1 DANs (schematised in Figure 9).
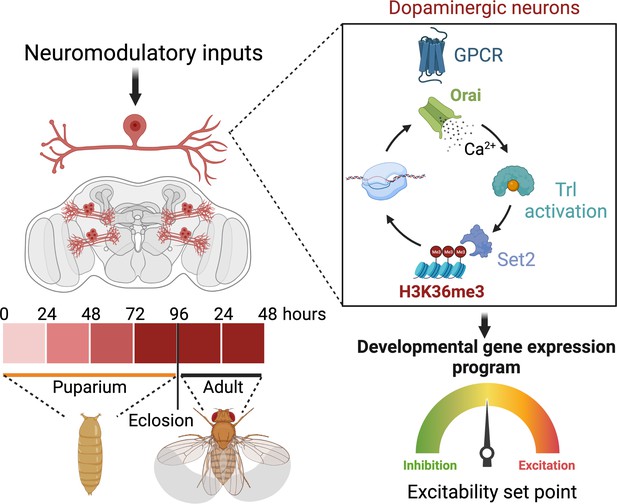
Schematic outline of the role of store-operated Ca2+ entry (SOCE) in determining excitability and function of dopaminergic neurons required for flight circuit development.
SOCE acts through a transcriptional feedback loop including the transcription factor trithorax-like (Trl), and the H3K36 methyltransferase Set2 to induce the expression of genes required for neuronal excitability and flight.
SOCE supports gene expression transitions during critical developmental windows
Studies in the Drosophila MB have identified spontaneous bouts of voltage-gated Ca2+ channel-mediated neuronal activity through as yet unknown mechanisms, which occur during early adulthood driving refinement and maturation of behaviours such as associative learning (Leinwand and Scott, 2021). Our studies here, on a subset of 21–23 central DANs (Figure 1B and C) of which some send projections to the MB and regulate flight behaviour, provide a mechanistic explanation for neuromodulation-dependent intracellular signalling culminating in transcriptional maturation of a circuit supporting adult flight. In the critical developmental window of 72–96 hr APF, a cohort of SOCE-responsive genes, which include a range of ion channels, undergo a wave of induction. Loss of SOCE at this stage thus renders these neurons functionally incompetent (Figures 3B–D–6G–I, 7D–F) and abrogates flight (Figure 1D). Developmentally assembled neuronal circuits require experience/activity-dependent maturation (Akin and Zipursky, 2020). The requirement of SOCE for flight circuit function during the early post-eclosion phase (0–2 d) suggests that maturation of the flight circuit extends into early adulthood, where presumably feedback from circuit activity may further refine synaptic strengths (Sugie et al., 2018). Expression of OraiE180A in 5-day-old adults had no effect on flight (Figure 1E), indicating that either SOCE is not required for maintaining gene expression in adults or that wildtype Orai channel proteins from late pupal and early adults perdure for long periods extending into adulthood. Loss of SOCE in adult brains beyond 5–7 d, when Orai protein turnover might be expected, has not been assessed so far. The absence of any visible changes to primary neurite patterning suggests that SOCE in the MB-DANs works primarily to refine synaptic function at presynaptic terminals within the MB lobe. Taken together, our findings identify a role for SOCE in restructuring the neuronal transcriptome during a critical developmental transition, which facilitates key changes in neuronal function required for circuit formation and adult behaviour (schematised in Figure 9). In summary, our findings here identify Orai-driven Ca2+ entry as a key signalling step for driving coordinated gene expression enabling the maturation of nascent neuronal circuits and sustenance of adult behaviour.
SOCE regulates a balance between competing epigenetic signatures
Chromatin structure and function are dynamic over the course of brain development (Kishi and Gotoh, 2018). One way neurons achieve dynamic spatio-temporal control over developmental gene expression is via epigenetic mechanisms such as post-translational histone modifications (Geng et al., 2021). Cells possess an extensive toolkit of histone modifiers, with characterised effects on transcriptional output by either activating or repressing gene expression (Bannister and Kouzarides, 2011). However, relatively little is known about how modifiers of histone marks are in turn regulated to bring about the requisite changes in neuronal gene expression over developmental timescales.
The SET-domain containing family of histone modifiers (Figure 2B), including H3K36me3 methyltransferase Set2 (Figure 2C), identified here appears to function as key transcriptional effectors-induced downstream of neuromodulatory inputs that stimulate SOCE through Orai during pupal and adult maturation of fpDANs. SOCE-driven Set2-mediated H3K36me3 enhances the expression of key GPCRS, components of intracellular Ca2+ signalling (Figure 2A) and ion channels (Figure 6A and B) for optimal dopaminergic neuron function (Figure 7D–F) and flight (Figure 1C). Rescue of flight by genetic depletion of an epigenetic ‘eraser’ Kdm4B, an H3K36me3 demethylase (Figure 2D), suggests that a balance between perdurance of H3K36me3 and its removal is actively maintained on the expressed genes. Interestingly, another member of the SET domain family, E(z), is upregulated upon loss of SOCE (Figure 2B). E(z) is a component of the Drosophila PRC2 complex, which represses gene expression through H2K27me3 (Margueron and Reinberg, 2011). Structural studies on Drosophila nucleosomes have elucidated that the H3K36me3 modification allosterically inhibits the PRC2 complex activity (Finogenova et al., 2020). Our findings support a model wherein at key developmental stages SOCE determines the level of these competing epigenetic signatures, thus allowing gene expression by enhancing Set2-mediated H3K36me3 (Figure 2H). Loss of SOCE leads to deficient Set2-mediated H3K36me3 (Figure 2E), a likely shifting of the balance in favour of PRC2-mediated H3K27me3 and overall repression in gene expression (as observed in Figure 1—figure supplements 8 and 9). Feeding SOCE-deficient animals a pharmacological inhibitor of PRC2 (GSK434) led to a significant rescue in behaviour (Figure 2J) presumably by disinhibition of H3K27me3-mediated gene repression. Though flight initiation is rescued in a dose-dependent manner, the duration of flight bouts is not sustained (Figure 2—figure supplement 8), indicating that suppression of H3K27me3 marks in the SOCE-deficient flies is partial. These findings indicate additional roles for other histone modifications such as Set1-mediated H3K4me3, which may work in concert to regulate developmental gene expression programs stimulated by SOCE. Future studies directed at a comprehensive cataloguing of multiple epigenetic signatures in defined neuronal subsets using cell-specific techniques should help elucidate these mechanisms.
Trl as an SOCE-responsive transcription factor
SOCE-mediated regulation of gene expression has been reported in mammalian neural progenitor cells (Somasundaram et al., 2014) and Drosophila pupal neurons (Pathak et al., 2015; Richhariya et al., 2017), through as yet unknown mechanisms. A combination of motif enrichment analysis over regulatory regions of SOCE-responsive genes and expression enrichment analysis in cells of interest helped generate a list of putative SOCE-responsive TFs (Figure 4A and B). Among these, we experimentally validated Trl/trithorax-like/GAGA factor (GAF) as a TF required for maturation of excitability in fpDANs using genetic tools (Figure 4). We found that loss of Trl in the fpDANs results in significant flight deficits, which could be partially rescued through excess STIM (which raises SOCE). This indicates that increased Ca2+ entry through Orai either activates residual Trl or alternates SOCE-responsive TFs to induce Set2 expression and rescue downstream gene expression essential for flight.
Although Trl function has been reported in the context of early embryonic development, where it has been implicated in zygotic genome activation (Gaskill et al., 2021) (ZGA), expression of Hox genes (Shimojima et al., 2003), dosage compensation (Greenberg et al., 2004), and expression of a Drosophila voltage-gated calcium channel subunit in germ cell development (Dorogova et al., 2014), this is the first ever report of its role in regulating neuronal gene expression in the context of SOCE and circuit maturation. The molecular mechanism by which Orai-mediated Ca2+ entry leads to activation of Trl needs further elucidation. Our finding that CaMKII hyperactivation partially rescues flight deficits caused by loss of Orai function, taken together with the bioinformatic prediction for CaMKII-mediated Trl phosphorylation, needs to be experimentally verified. Future studies could be directed towards looking at the interactions between these two proteins using in vitro biochemical assays.
In vitro studies on Drosophila Trl reveal that it utilises a Q-rich intrinsically disordered domain (IDD) to self-multimerise or interact with a wide range of accessory proteins (Wilkins and Lis, 1999). Multimeric complexes of Trl reside on chromatin with an exceptionally long residence time and maintain chromatin in an ‘open’ state (Tang et al., 2022). Trl has also been reported to directly interact with components of the transcription initiation and elongation complex (Chopra et al., 2008; Li et al., 2013), support long-distance promoter–enhancer interactions (Mahmoudi et al., 2003), mediate active ATP-dependent chromatin remodelling by maintaining nucleosome-free stretches (Tsukiyama et al., 1994), and regulate global gene expression by controlling transcriptional stalling and pausing (Tsai et al., 2016). A recent study demonstrates a role for Trl in chromatin folding in Drosophila neurons to enable cell type-specific gene expression (Mohana et al., 2023). SOCE-dependent gene regulation may rely upon multiple TFs acting in concert in a cell type and developmental stage-specific contexts, of which Trl may be just one. Future studies directed at other possible TFs downstream of SOCE would be of interest.
SOCE and the control of neuronal activity and cholinergic neuromodulation of a tripartite synapse in the MB
Neurons undergo maturation of their electrical properties with a gradual increase in depolarising responses and synaptic transmission over the course of pupal development (Järvilehto and Finell, 1983). Here, we show that a subset of DANs require a balance of ion channels for optimal excitation and inhibition essential for adult function. Neuromodulatory signals, such as acetylcholine, are essential for acquiring this balance and act via an SOCE-Trl-Set2 mechanism during late pupal and early adult stages. The ability of Set2 overexpression to rescue SOCE-deficient phenotypes (Figure 6A–C) indicates that anomalous gene expression is the underlying basis of this phenotype. Indeed, SOCE-deficient DANs show reduced expression of an ensemble of voltage-gated ion channel genes (Figure 5E and F), including genes encoding the outward-rectifying K+ channel shaw, slow-inactivating voltage-gated K+ channel shaker, and a Ca2+-gated K+ channel slowpoke and cacophony, a subunit of the voltage-gated Ca2+ channel (Figure 6G). Ion channels like Shaker and slowpoke play an important role in establishing resting membrane potential (Singh and Wu, 1990), neuronal repolarisation (Lichtinghagen et al., 1990), and mediating afterhyperpolarisation (AHP) after repeated bouts of activity (Ping et al., 2011). Though DANs require Set2-mediated expression of cacophony for excitability, cacophony is in itself insufficient to rescue the loss in excitability and flight deficits upon loss of SOCE (Figure 6J). Future experiments that directly visualise electrophysiological responses of fpDANs from different genotypes would help resolve the role of Cac and other ion channels and determine their individual contributions to THD’ neuronal activity and excitability. Both flight and KCl depolarisation in SOCE-deficient neurons could, however, be rescued by expression of a heterologous depolarisation-activated Na+ channel NachBac (Nitabach et al., 2006; Figure 7B–D), which increases neuronal excitability by stimulating a low-threshold positive feedback loop and to a lesser extent by CsChrimson-mediated optogenetic induction of neuronal activity (Figure 7E). The poorer rescue by optogenetic activation may stem from the fact that sustained bursts of activity are non-physiological and can deplete the readily releasable pool of neurotransmitters (Arrigoni and Saper, 2014; Kaeser and Regehr, 2017). Other indirect forms of inducing neuronal hyperactivation (Figure 7—figure supplement 1) in SOCE-deficient neurons also achieved minor rescues in flight. These results identify SOCE as a key driver downstream of developmentally salient neuromodulatory signals for expression of an ion channels suite that enables generation of intrinsic electrical properties and functional maturation of the PPL1 DANs and the flight circuit.
Limitations of this study
Direct measurements of SOCE are generally performed in cultured cells by depletion of ER-store Ca2+ in ‘0’ Ca2+ medium, followed by Ca2+-add back to measure SOCE. In this study, we were unable to perform similar SOCE measurements from the fpDANs because they consist of 16–19 neurons in each hemisphere (PPL1 are 10–12 and PPM3 are 6–7 cells; Pathak et al., 2015) and identifying these few neurons was not technically feasible in culture. Measuring SOCE from these neurons in vivo was not possible due to the presence of abundant extracellular Ca2+ in the brain. Due to these reasons, we have relied upon using CCh to elicit IP3-mediated Ca2+ release and SOCE as a proxy for in vivo SOCE. In previous studies, we have shown that CCh treatment of cultured Drosophila neurons elicits IP3-mediated Ca2+ release and SOCE (Agrawal et al., 2010). Moreover, expression of OraiE180A completely blocks SOCE as measured in primary cultures of DANs (Pathak et al., 2015) and CCh-induced IP3-mediated Ca2+ release is tightly coupled to SOCE in Drosophila neurons (Venkiteswaran and Hasan, 2009; Chakraborty et al., 2016; Chakraborty and Hasan, 2017). We posit that our measurements of CCh-evoked changes in cellular Ca2+ reflect a composite of IP3-mediated Ca2+ release and SOCE.
Although we have provided compelling genetic evidence for Trl as an SOCE-responsive TF, the detailed biochemical basis of Trl activation was beyond the scope of this study. We propose phosphorylation by CamKII as a possible mechanism that needs further investigation.
Materials and methods
Fly maintenance
Request a detailed protocolDrosophila strains were grown on standard cornmeal medium consisting of 80 g corn flour, 20 g glucose, 40 g sugar, 15 g yeast extract, 4 ml propionic acid, 5 ml p-hydroxybenzoic acid methyl ester in ethanol, and 5 ml ortho-butyric acid in a total volume of 1 l (ND) at 25°C under a light–dark cycle of 12 hr and 12 hr. Canton S was used as wildtype throughout. Mixed sex populations were used for all experiments. Several fly stocks used in this study were sourced using FlyBase (https://flybase.org), which is supported by a grant from the National Human Genome Research Institute at the US National Institutes of Health (#U41 HG000739), the British Medical Research Council (#MR/N030117/1), and FlyBase users from across the world. The stocks were obtained from the Bloomington Drosophila Stock Centre (BDSC) supported by NIH P40OD018537. All fly stocks used and their sources are listed in Supplementary file 1b.
Flight assays
Request a detailed protocolFlight assays were performed as previously described (Manjila and Hasan, 2018). Briefly, flies aged 3–5 d of either sex were tested in batches of 8–10 flies, and a minimum of 30 flies were tested for each genotype. Adult flies were anaesthetised on ice for 2–3 min and then tethered between at the head–thorax junction using a thin metal wire and nail polish. Post recovery at room temperature for 2–3 min, an air puff was provided as stimulus to initiate flight. Flight duration was recorded for each fly for 15 min. For all control genotypes, GAL4 or UAS strains were crossed to the wildtype strain, Canton S. Flight assays are represented in the form of a swarm plot, wherein each dot represents a flight bout duration for a single fly. The colours indicate different genotypes. The Δ Flight parameter refers to the mean difference for comparisons against the shared CS control which is shown as a Cumming estimation plot (Ho et al., 2018). On the lower axes, mean differences are plotted as bootstrap sampling distributions. Each mean difference is depicted as a dot. Each 95% confidence interval is indicated by the ends of the vertical error bars. Letters beneath each distribution refer to statistically indistinguishable groups after performing a Kruskal–Wallis test followed by a post hoc Mann–Whitney U-test (p<0.05).
FACS
Request a detailed protocolFluorescence-activated cell sorting (FACS) was used to enrich eGFP-labelled DANs from pupal/adult. The following genotypes were used for sorting: wildtype (THD’GAL4>UAS-eGFP), OraiE180A (THD’GAL4>OraiEE180A), Set2IR-1(THD’GAL4>Set2IR-1), OraiE180A; Set2OE (THD’GAL4>OraiEE180A; Set2OE), Set2OE (THD’GAL4>Set2OE), and TrlIR-1 (THD’GAL4>TrlIRIR-1). Approximately 100 pupae per sample were washed in 1× PBS and 70% ethanol. Pupal/adult CNSs were dissected in Schneider’s medium (Thermo Fisher Scientific) supplemented with 10% fetal bovine serum, 2% PenStrep, 0.02 mM insulin, 20 mM glutamine, and 0.04 mg/ml glutathione. Post dissection, the CNSs were treated with an enzyme solution (0.75 g/l collagenase and 0.4 g/l dispase in Rinaldini’s solution [8 mg/ml NaCl, 0.2 mg/ml KCl, 0.05 mg/ml NaH2PO4, 1 mg/ml NaHCO3, and 0.1 mg/ml glucose]) at room temperature for 30 min. They were then washed and resuspended in ice-cold Schneider’s medium and gently triturated several times using a pipette tip to obtain a single-cell suspension. This suspension was then passed through a 40 mm mesh filter to remove clumps and kept on ice until sorting (less than 1 hr). Flow cytometry was performed on a FACS Aria Fusion cell sorter (BD Biosciences) with a 100 mm nozzle at 60 psi. The threshold for GFP-positive cells was set using dissociated neurons from a non-GFP-expressing wildtype strain (Canton S). The same gating parameters were used to sort other genotypes in the experiment. GFP-positive cells were collected directly in TRIzol and then frozen immediately in dry ice until further processing. Details for all reagents used are listed in Supplementary file 1b.
RNA-seq
Request a detailed protocolRNA from at least 600 sorted THD’ DANs from the relevant genotypes, expressing UAS-eGFP, was subjected to 14 cycles of PCR amplification (SMARTer Seq V4 Ultra Low Input RNA Kit; Takara Bio). Then, 1 ng of amplified RNA was used to prepare cDNA libraries (Nextera XT DNA library preparation kit; Illumina). cDNA libraries for four biological replicates for both control (THD’GAL4/+) and experimental (THD’GAL4>UAS-OraiE180A) genotypes were run on a Hiseq2500 platform. Then, 50–70 million unpaired sequencing reads per sample were aligned to the dm6 release of the Drosophila genome using HISAT2 (Kim et al., 2015; Kim et al., 2019) and an overall alignment rate of 95.2–96.8% was obtained for all samples. Featurecounts (Liao et al., 2014) was used to assign the mapped sequence reads to the genome and obtain read counts. Differential expression analysis was performed using three independent methods: DESeq2 (Love et al., 2014), limma-voom (Ritchie et al., 2015), and edgeR (Robinson et al., 2010). A fold change cut-off of a minimum twofold change was used. Significance cut-off was set at an FDR-corrected p-value of 0.05 for DESeq2 and edgeR. Volcanoplots were generated using VolcaNoseR (https://huygens.science.uva.nl/). Comparison of gene lists and generation of Venn diagrams was performed using Whitehead BaRC public tools (http://jura.wi.mit.edu/bioc/tools/).GO analysis for molecular function was performed using DAVID (Huang et al., 2009). Developmental gene expression levels were measured for downregulated genes using FlyBase (Larkin et al., 2021) and DGET (Hu et al., 2017; https://www.flyrnai.org/tools/dget/web/) and were plotted as a heatmap using ClustVis (Metsalu and Vilo, 2015; https://biit.cs.ut.ee/clustvis/).
qRT-PCRs
Request a detailed protocolCNSs of the appropriate genotype and age were dissected in 1× phosphate-buffered saline (PBS; 137 mM NaCl, 2.7 mM KCl, 10 mM Na2HPO4, and 1.8 mM KH2PO4) prepared in double-distilled water treated with DEPC. Each sample consisted of five CNS homogenised in 500 µl of TRIzol (Ambion, Thermo Fisher Scientific) per sample. At least three biological replicate samples were made for each genotype. After homogenisation, the sample was kept on ice and either processed further within 30 min or stored at −80°C for up to 4 wk before processing. RNA was isolated following the manufacturer’s protocol. Purity of the isolated RNA was estimated by NanoDrop spectrophotometer (Thermo Fisher Scientific) and integrity was checked by running it on a 1% Tris-EDTA agarose gel. Approximately 100 ng of total RNA was used per sample for cDNA synthesis. DNAse treatment and first-strand synthesis were performed as described previously (Pathak et al., 2015). Quantitative real-time PCRs (qPCRs) were performed in a total volume of 10 µl with Kapa SYBR Fast qPCR kit (KAPA Biosystems) on an ABI 7500 fast machine operated with ABI 7500 software (Applied Biosystems). Technical duplicates were performed for each qPCR reaction. The fold change of gene expression in any experimental condition relative to wildtype was calculated as 2−ΔΔCt, where ΔΔCt = [Ct (target gene) –Ct (rp49)] Expt. – [Ct (target gene) – Ct (rp49)]. Primers specific for rp49 and ac5c were used as internal controls. Sequences of all primers used are provided in Table S1c.
Functional imaging
Request a detailed protocolAdult brains were dissected in adult hemolymph-like saline (AHL: 108 mM NaCl, 5 mM KCl, 2 mM CaCl2, 8.2 mM MgCl2, 4 mM NaHCO3, 1 mM NaH2PO4, 5 mM trehalose, 10 mM sucrose, 5 mM Tris, pH 7.5) after embedded in a drop of 0.1% low-melting agarose (Invitrogen). Embedded brains were bathed in AHL and then subjected to functional imaging. The genetically encoded calcium sensor GCaMP6m (Chen et al., 2013) was used to record changes in intracellular cytosolic Ca2+ in response to stimulation with CCh or KCl. The GRAB-DA sensor (Sun et al., 2018) was used to measure evoked dopamine release. Images were taken as a time series on an xy plane at an interval of 1 s using a ×20 objective with an NA of 0.7 on an Olympus FV3000 inverted confocal microscope. A 488 nm laser line was used to record GCaMP6m/GRAB-DA measurements. All live-imaging experiments were performed with at least 10 independent brain preparations. For measuring evoked responses, KCl/CCh was added on top of the samples. For optogenetics experiments, flies were reared on medium supplemented with 200 μM all trans retinal (ATR), following which neuronal activation was achieved using a 633 nm laser line to stimulate CsChrimson (Klapoetke et al., 2014) while simultaneously acquiring images with the 488 nm laser line, and the images were acquired every 1 s. The raw images were extracted using FIJI (Schindelin et al., 2012; based on ImageJ version 2.1.0/1.53c). ΔF/F was calculated from selected regions of interest (ROIs) using the formula ΔF/F = (Ft – F0)/F0, where Ft is the fluorescence at time t and F0 is baseline fluorescence corresponding to the average fluorescence over the first 40 time frames. Mean ΔF/F time lapses were plotted using PlotTwist (Goedhart, 2020; https://huygens.science.uva.nl/PlotTwist/). A shaded error bar around the mean indicates the 95% confidence interval for CCh (50 µM) or KCl (70 mM) responses. Peaks for individual cellular responses for each genotype were calculated from before or after the point of stimulation using Microsoft Excel and plotted as a paired plot using SuperPlotsOfData (Goedhart and Pollard, 2021; https://huygens.science.uva.nl/SuperPlotsOfData/). Boxplots were plotted using PlotsOfData (Postma and Goedhart, 2019; https://huygens.science.uva.nl/PlotsOfData/).
Immunohistochemistry
Request a detailed protocolImmunostaining of larval Drosophila brains was performed as described previously (Daniels et al., 2008). Briefly, adult brains were dissected in 1× PBS and fixed with 4% paraformaldehyde. The dissected brains were washed 3–4 times with 0.2% phosphate buffer, pH 7.2 containing 0.2% Triton-X 100 (PTX), and blocked with 0.2% PTX containing 5% normal goat serum (NGS) for 2 hr at room temperature. Respective primary antibodies were incubated overnight (14–16 hr) at 4°C. After washing 3–4 times with 0.2% PTX at room temperature, they were incubated in the respective secondary antibodies for 2 hr at room temperature. The following primary antibodies were used: chick anti-GFP antibody (1:10,000; A6455, Life Technologies), mouse anti-nc82 (anti-brp) antibody (1:50), rabbit anti-H3K36me3, and mouse anti-RFP. Secondary antibodies were used at a dilution of 1:400 as follows: anti-rabbit Alexa Fluor 488 (#A11008, Life Technologies) and anti-mouse Alexa Fluor 488 (#A11001, Life Technologies). Confocal images were obtained on the Olympus Confocal FV1000 microscope (Olympus) with a ×40, 1.3 NA objective or with a ×60, 1.4 NA objective. Imaging for axonal arbors within the MB γ lobe was performed on the Zeiss LSM980 system with AiryScan 2. Images were visualised using either the FV10-ASW 4.0 viewer (Olympus) or FIJI (Schindelin et al., 2012). Details for all reagents used are listed in Table S1b.
Western blotting
Request a detailed protocolAdult CNSs of appropriate genotypes were dissected in ice-cold PBS. Between 5 and 10 brains were homogenised in 50 μl of NETN buffer (100 mM NaCl, 20 mM Tris–Cl [pH 8.0], 0.5 mM EDTA, 0.5% Triton-X-100, 1× Protease inhibitor cocktail [Roche]). The homogenate (10–15 μl) was run on an 8% SDS-polyacrylamide gel. The protein was transferred to a PVDF membrane by standard semi-dry transfer protocols (10 V for 10 min). The membrane was incubated in the primary antibody overnight at 4°C. Primary antibodies were used at the following dilutions: rabbit anti-H3K36me3 (1:5000, Abcam, ab9050) and rabbit anti-H3 (1:5000, Abcam, ab12079). Secondary antibodies conjugated with horseradish peroxidase were used at dilution of 1:3000 (anti-rabbit HRP; 32260, Thermo Scientific). Protein was detected by a chemiluminescent reaction (WesternBright ECL, Advansta K12045-20). Blots were first probed for H3K36me3, stripped with a solution of 3% glacial acetic acid for 10 min, followed by re-probing with the anti-H3 antibody.
In silico ChIP-seq analysis
Request a detailed protocolH3K36me3 enrichment data was obtained from a ChIP-chip dataset (ID_301) generated in Drosophila ML-DmBG3-c2 cells submitted to modEncode (Celniker et al., 2009; modENCODE Consortium et al., 2010). Enrichment scores for genomic regions were calculated using ‘computematrix’ and plotted as a tag density plot using ‘plotHeatmap’ from deeptools2 (Ramírez et al., 2016). All genes were scaled to 2 kb with a flanking region of 250 bp on either end. A 50 bp length of non-overlapping bins was used for averaging the score over each region length. Genes were sorted based on mean enrichment scores and displayed on the heatmap in descending order.
Statistical tests
Request a detailed protocolNon-parametric tests were employed to test significance for data that did not follow a normal distribution. Significant differences between experimental genotypes and relevant controls were tested either with the Kruskal–Wallis test followed by Dunn’s multiple-comparison test (for multiple comparisons) or with Mann–Whitney U-tests (for pairwise comparisons). Data with normal distribution were tested by Student’s t-test. Statistical tests and p-values are mentioned in each figure legend. For calculation and representation of effect size, estimationstats.com was used.
Data availability
Sequencing data have been deposited in GEO under accession codes GSE2301342. Source data for each figure and figure supplements have been provided. All reagent information has been listed. A list of all primer sequences used and Drosophila stocks has been provided.
-
NCBI Gene Expression OmnibusID GSE230134. Orai-mediated calcium entry regulates the excitability of central dopaminergic neurons.
-
NCBI Gene Expression OmnibusID GSE47280. H3K36me3 abcam.OR Head Nuclei.Solexa.
-
NCBI Gene Expression OmnibusID GSE47319. H3K27me3 (Abcam lot3).OR Head Nuclei.Solexa.
-
NCBI BioProjectID PRJNA75285. BDGP modENCODE D. melanogaster Transcriptome Sequencing.
References
-
A genetic RNAi screen for IP₃/CaPLOS Genetics 9:e1003849.https://doi.org/10.1371/journal.pgen.1003849
-
Activity regulates brain development in the flyCurrent Opinion in Genetics & Development 65:8–13.https://doi.org/10.1016/j.gde.2020.04.005
-
Regulation of chromatin by histone modificationsCell Research 21:381–395.https://doi.org/10.1038/cr.2011.22
-
Transcriptional activation by GAGA factor is through its direct interaction with dmTAF3Developmental Biology 317:660–670.https://doi.org/10.1016/j.ydbio.2008.02.008
-
Visualizing glutamatergic cell bodies and synapses in Drosophila larval and adult CNSThe Journal of Comparative Neurology 508:131–152.https://doi.org/10.1002/cne.21670
-
The histone modifications of neuronal plasticityNeural Plasticity 2021:6690523.https://doi.org/10.1155/2021/6690523
-
Regulation of neuronal physiology by Ca2+ release through the IP3RCurrent Opinion in Physiology 17:1–8.https://doi.org/10.1016/j.cophys.2020.06.001
-
Transcriptional regulation by calcium, calcineurin, and NFATGenes & Development 17:2205–2232.https://doi.org/10.1101/gad.1102703
-
Development of the function of visual receptor cells during the pupal life of the flyCalliphoraJournal of Comparative Physiology? A 150:529–536.https://doi.org/10.1007/BF00609579
-
Inducible genetic suppression of neuronal excitabilityThe Journal of Neuroscience 19:1691–1697.https://doi.org/10.1523/JNEUROSCI.19-05-01691.1999
-
The readily releasable pool of synaptic vesiclesCurrent Opinion in Neurobiology 43:63–70.https://doi.org/10.1016/j.conb.2016.12.012
-
The Drosophila NFAT homolog is involved in salt stress toleranceInsect Biochemistry and Molecular Biology 37:356–362.https://doi.org/10.1016/j.ibmb.2006.12.009
-
HISAT: a fast spliced aligner with low memory requirementsNature Methods 12:357–360.https://doi.org/10.1038/nmeth.3317
-
Graph-based genome alignment and genotyping with HISAT2 and HISAT-genotypeNature Biotechnology 37:907–915.https://doi.org/10.1038/s41587-019-0201-4
-
Regulation of chromatin structure during neural developmentFrontiers in Neuroscience 12:874.https://doi.org/10.3389/fnins.2018.00874
-
Independent optical excitation of distinct neural populationsNature Methods 11:338–346.https://doi.org/10.1038/nmeth.2836
-
Methylation of histone H3 by Set2 in Saccharomyces cerevisiae is linked to transcriptional elongation by RNA polymerase IIMolecular and Cellular Biology 23:4207–4218.https://doi.org/10.1128/MCB.23.12.4207-4218.2003
-
FlyBase: updates to the Drosophila melanogaster knowledge baseNucleic Acids Research 49:D899–D907.https://doi.org/10.1093/nar/gkaa1026
-
Changes in neuronal circuits during insect metamorphosisThe Journal of Experimental Biology 112:27–44.https://doi.org/10.1242/jeb.112.1.27
-
GAGA facilitates binding of Pleiohomeotic to a chromatinized Polycomb response elementNucleic Acids Research 31:4147–4156.https://doi.org/10.1093/nar/gkg479
-
CASK and CaMKII function in Drosophila memoryFrontiers in Neuroscience 8:178.https://doi.org/10.3389/fnins.2014.00178
-
Neuronal excitability as a regulator of circuit remodelingCurrent Biology 33:981–989.https://doi.org/10.1016/j.cub.2023.01.032
-
Store-operated Ca2+ entry regulates neuronal gene expression and functionCurrent Opinion in Neurobiology 73:102520.https://doi.org/10.1016/j.conb.2022.01.005
-
Store-operated calcium entry through orai is required for transcriptional maturation of the flight circuit in DrosophilaThe Journal of Neuroscience 35:13784–13799.https://doi.org/10.1523/JNEUROSCI.1680-15.2015
-
Store-operated calcium channelsPhysiological Reviews 95:1383–1436.https://doi.org/10.1152/physrev.00020.2014
-
deepTools2: a next generation web server for deep-sequencing data analysisNucleic Acids Research 44:W160–W165.https://doi.org/10.1093/nar/gkw257
-
Punishment prediction by dopaminergic neurons in DrosophilaCurrent Biology 15:1953–1960.https://doi.org/10.1016/j.cub.2005.09.042
-
Calcium signaling in neuronal developmentCold Spring Harbor Perspectives in Biology 3:a004259.https://doi.org/10.1101/cshperspect.a004259
-
Fiji: an open-source platform for biological-image analysisNature Methods 9:676–682.https://doi.org/10.1038/nmeth.2019
-
Differential control of presynaptic CaMKII activation and translocation to active zonesThe Journal of Neuroscience 31:9093–9100.https://doi.org/10.1523/JNEUROSCI.0550-11.2011
-
Properties of potassium currents and their role in membrane excitability in Drosophila larval muscle fibersThe Journal of Experimental Biology 152:59–76.https://doi.org/10.1242/jeb.152.1.59
-
Store-operated CRAC channels regulate gene expression and proliferation in neural progenitor cellsThe Journal of Neuroscience 34:9107–9123.https://doi.org/10.1523/JNEUROSCI.0263-14.2014
-
Activity-dependent neurotransmitter respecificationNature Reviews. Neuroscience 13:94–106.https://doi.org/10.1038/nrn3154
-
Drosophila dSet2 functions in H3-K36 methylation and is required for developmentBiochemical and Biophysical Research Communications 359:784–789.https://doi.org/10.1016/j.bbrc.2007.05.189
-
Effect of inositol-1,4,5-trisphosphate on isolated subcellular fractions of rat pancreasThe Journal of Membrane Biology 81:241–253.https://doi.org/10.1007/BF01868717
-
Volume transmission in the brain: beyond the synapseThe Journal of Neuropsychiatry and Clinical Neurosciences 26:13110351.https://doi.org/10.1176/appi.neuropsych.13110351
-
Kinetic principles underlying pioneer function of GAGA transcription factor in live cellsNature Structural & Molecular Biology 29:665–676.https://doi.org/10.1038/s41594-022-00800-z
-
IP3 receptors and Ca2+ entryBiochimica et Biophysica Acta 1866:1092–1100.https://doi.org/10.1016/j.bbamcr.2018.11.007
-
Neurobiology: setting the set point for neural homeostasisCurrent Biology 25:R1132–R1133.https://doi.org/10.1016/j.cub.2015.10.021
-
ConferenceIntracellular Ca2+ signalling and store operated Ca2+ entry are required in Drosophila neurons for flightProceedings of the National Academy of Sciences.
-
Identification of potent, selective, cell-active inhibitors of the histone lysine methyltransferase EZH2ACS Medicinal Chemistry Letters 3:1091–1096.https://doi.org/10.1021/ml3003346
-
Neural plasticity: dopamine tunes the mushroom body output networkCurrent Biology 26:R109–R112.https://doi.org/10.1016/j.cub.2015.12.023
-
DNA distortion and multimerization: novel functions of the glutamine-rich domain of GAGA factorJournal of Molecular Biology 285:515–525.https://doi.org/10.1006/jmbi.1998.2356
-
Phosphoproteome analysis of Drosophila melanogaster embryosJournal of Proteome Research 7:1675–1682.https://doi.org/10.1021/pr700696a
Article and author information
Author details
Funding
Department of Biotechnology, Ministry of Science and Technology, India (BT/PR28450/MED/122/2018)
- Gaiti Hasan
The funders had no role in study design, data collection and interpretation, or the decision to submit the work for publication.
Acknowledgements
We acknowledge the Central Imaging and Flow Cytometry Facility (CIFF, NCBS) for maintenance of microscopes and the Drosophila facility (Flyfacility, NCBS) for Fly stock maintenance and development of transgenics. We thank Nandashree KS for helping with super-resolution imaging on the Zeiss 980 Airyscan system. This work was funded by grant no. BT/PR28450/MED/122/166/2018 from the Department of Biotechnology, Govt. of India, and core support by NCBS, TIFR. RM and SR received graduate student fellowships from NCBS, TIFR.
Ethics
This study involved invertebrates (Drosophila melanogaster) and was performed in strict accordance with institutional biosafety and animal handling guidelines.
Version history
- Sent for peer review:
- Preprint posted:
- Reviewed Preprint version 1:
- Reviewed Preprint version 2:
- Reviewed Preprint version 3:
- Version of Record published:
Cite all versions
You can cite all versions using the DOI https://doi.org/10.7554/eLife.88808. This DOI represents all versions, and will always resolve to the latest one.
Copyright
© 2023, Mitra et al.
This article is distributed under the terms of the Creative Commons Attribution License, which permits unrestricted use and redistribution provided that the original author and source are credited.
Metrics
-
- 1,421
- views
-
- 101
- downloads
-
- 2
- citations
Views, downloads and citations are aggregated across all versions of this paper published by eLife.
Download links
Downloads (link to download the article as PDF)
Open citations (links to open the citations from this article in various online reference manager services)
Cite this article (links to download the citations from this article in formats compatible with various reference manager tools)
Further reading
-
- Genetics and Genomics
N6-methyladenosine (m6A) in eukaryotic RNA is an epigenetic modification that is critical for RNA metabolism, gene expression regulation, and the development of organisms. Aberrant expression of m6A components appears in a variety of human diseases. RNA m6A modification in Drosophila has proven to be involved in sex determination regulated by Sxl and may affect X chromosome expression through the MSL complex. The dosage-related effects under the condition of genomic imbalance (i.e. aneuploidy) are related to various epigenetic regulatory mechanisms. Here, we investigated the roles of RNA m6A modification in unbalanced genomes using aneuploid Drosophila. The results showed that the expression of m6A components changed significantly under genomic imbalance, and affected the abundance and genome-wide distribution of m6A, which may be related to the developmental abnormalities of aneuploids. The relationships between methylation status and classical dosage effect, dosage compensation, and inverse dosage effect were also studied. In addition, we demonstrated that RNA m6A methylation may affect dosage-dependent gene regulation through dosage-sensitive modifiers, alternative splicing, the MSL complex, and other processes. More interestingly, there seems to be a close relationship between MSL complex and RNA m6A modification. It is found that ectopically overexpressed MSL complex, especially the levels of H4K16Ac through MOF, could influence the expression levels of m6A modification and genomic imbalance may be involved in this interaction. We found that m6A could affect the levels of H4K16Ac through MOF, a component of the MSL complex, and that genomic imbalance may be involved in this interaction. Altogether, our work reveals the dynamic and regulatory role of RNA m6A modification in unbalanced genomes, and may shed new light on the mechanisms of aneuploidy-related developmental abnormalities and diseases.
-
- Genetics and Genomics
The DYRK1A enzyme is a pivotal contributor to frequent and severe episodes of otitis media in Down syndrome, positioning it as a promising target for therapeutic interventions.