Ab initio prediction of specific phospholipid complexes and membrane association of HIV-1 MPER antibodies by multi-scale simulations
Figures
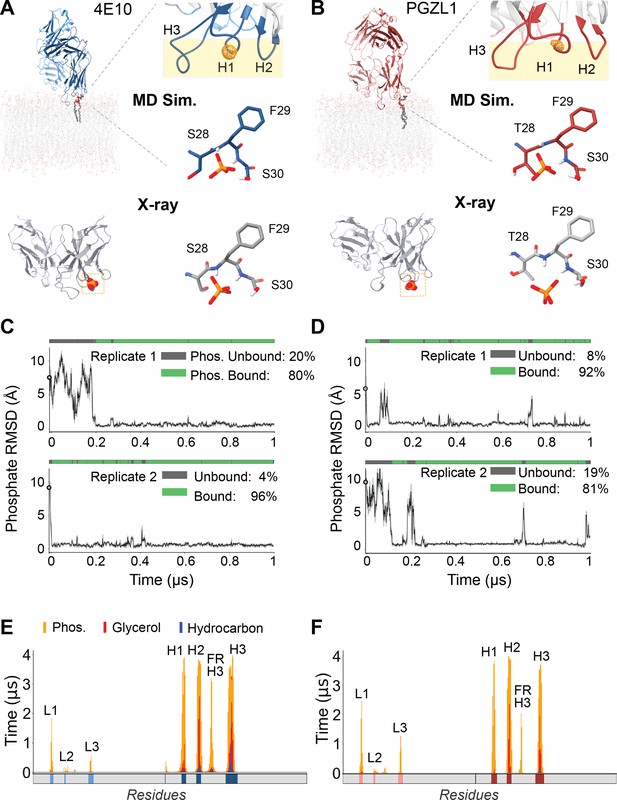
4E10 and PGZL1 CDR lipid phosphate binding sites and membrane interaction profiles in all-atom fluid HIV-like bilayer MD simulations.
(A) Representative frame from an MD simulation of the phospholipid complex at 4E10 Fab CDR-H1 (top left). Top right, time-averaged lipid phosphate density (orange mesh) relative to antibody CDR loops embedded in the bilayer (beige) from 4 µs total time (top right). Center right, CDR-H1 loop side chain and backbone atoms of de novo predicted phosphate interaction (middle right). Bottom, putative lipid phosphate binding site observed in an X-ray structure for 4E10 (PDB: 4XC1), comparing the CDR-H1 loop interactions to MD (bottom right). (B) PGZL1 lipid phosphate interaction at the CDR-H1 loop from MD simulation versus X-ray crystallography (PDB: 6O3J), demarked as in (A). (C) RMSD of the interacting lipid phosphate versus the experimental CDR-phosphate position (X-ray site), classified as bound (green) and unbound (grey) by loop-phosphate contacts (see Methods) in 2, 4E10 representative replicate trajectories. Black line, ten-frame RMSD running average; standard deviation, grey shading. (D) RMSD of lipid phosphate binding to PGZL1 CDR-H1 in MD simulations versus X-ray site. Phosphate binding is mapped above each MD trajectory as in (C). (E) Per-residue interaction profiles for Fab simulations of 4E10 detailing the time spent for each residue in in phosphate layer (orange), glycerol layer (red), or hydrocarbon layer (blue) across aggregate 4 µs from four simulations. CDR loops are mapped in solid color blocks below each profile. Fab domain regions making significant contact are labeled, including CDRs and heavy chain framework region 3 (FR-H3). (F) Per-residue interaction profiles for antibody Fab simulations for PGZL1, colored as in (E).
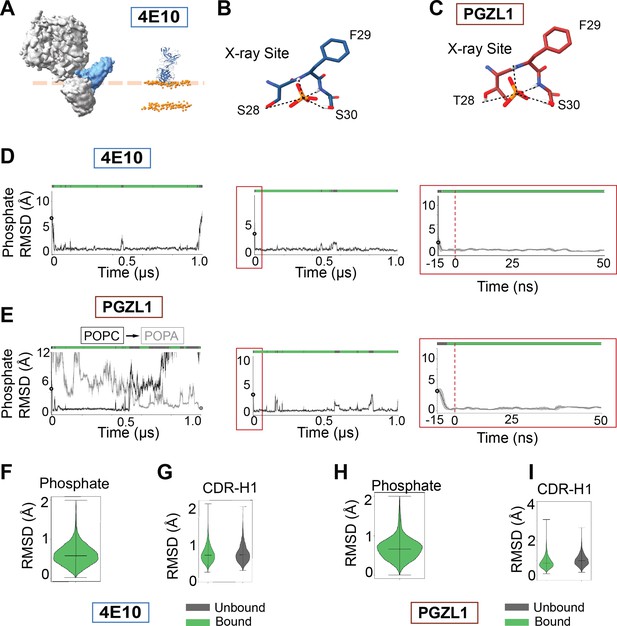
All atom MD replicates for 4E10 and PGZL1 with phosphate group interactions.
(A) Expected orientation of titled full-length HIV Env with one 4E10 Fab bound (EMD: 25024) from the membrane position inferred from the cryoEM density map compared to the initial docked orientation of 4E10 Fab from PPM2.0 used for simulation. (B) A phosphate was defined as ‘bound’ by defining the relative position after aligning 4E10 CDR-H1 backbone conformation of each simulation frame with that of the X-ray structure: relative distance of the phosphate atom of <2.0 Å the position of the phosphate in the crystallographic loop. As well, to control for potential backbone fluctuations, we also considered nearby positioning of at least two of the original hydrogen bond donor side chain or backbone donors within 5.25 Å to the central phosphate – accounting for the P-O bond length plus the distance of a weak hydrogen bond. (C) A phosphate was defined as bound in the PGZL1 CDR-H1 loop with the same distance metrics as detailed in (A) for 4E10. (D) Two additional replicates of 4E10 bnAb Fab all-atom MD simulations tracking reproducible lipid phosphate binding events. Right, for one replicate binding occurs as early as the preceding 15 ns NPT equilibration simulation where the protein structure is restrained but lipids can freely diffuse. The bound phospholipid-CDR complex is stable through its 1 µs production simulation. (E) Left, PGZL1 bnAb Fab all-atom simulation replicate observing a replacement event. One lipid phosphate group binds at the known interaction site, then exchanges with the headgroup of second lipid within the course of 1 µs. Right, additional MD simulation replicate of PGZL1 where lipid phosphate is bound rapidly, during the restrained NPT stage of the simulation (middle, right). (F) RMSD per frame distribution of lipid phosphate headgroup position compared to X-ray structure positions during bound time, showing sub-Å accuracy to experiment. (G) RMSD per frame distribution of 4E10 CDR-H1 loop backbone coordinates versus averaged reference coordinates show the rigidity of the loop during phospholipid bound (green) and unbound (grey) time from 4 µs aggregated simulation time, showing sub-Å RMSF. (H) RMSD per frame distribution of PGZL1 CDR-H1 loop backbone coordinates versus averaged reference coordinates for 4 µs aggregated simulation time, showing sub-Å RMSF. (I) RMSD per frame distribution of lipid phosphate headgroup position compared to X-ray structure positions during bound time for PGZL1, showing sub-Å accuracy to experiment.
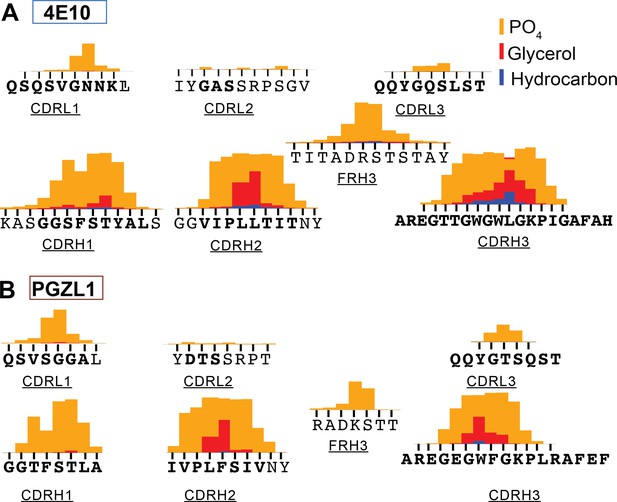
Detailed per-residue protein-lipid interaction analysis aggregated across simulations including primary sequence to fine 4E10 and PGZL1 bnAbs membrane-interacting region.
(A) Per-residue interaction profiles aligned with primary sequence for 4E10 CDR and framework regions, highlighting all residues which embed or peripherally contact lipids, defined by layers of different membrane depth and chemical environment, colored as in Figure 1E. Amino acids specifically within loops regions are bolded, with flanking membrane-contacting amino acid segments included for context. (B) Per-residue interaction profiles for lipid-interacting PGZL1 CDR loops and framework regions.
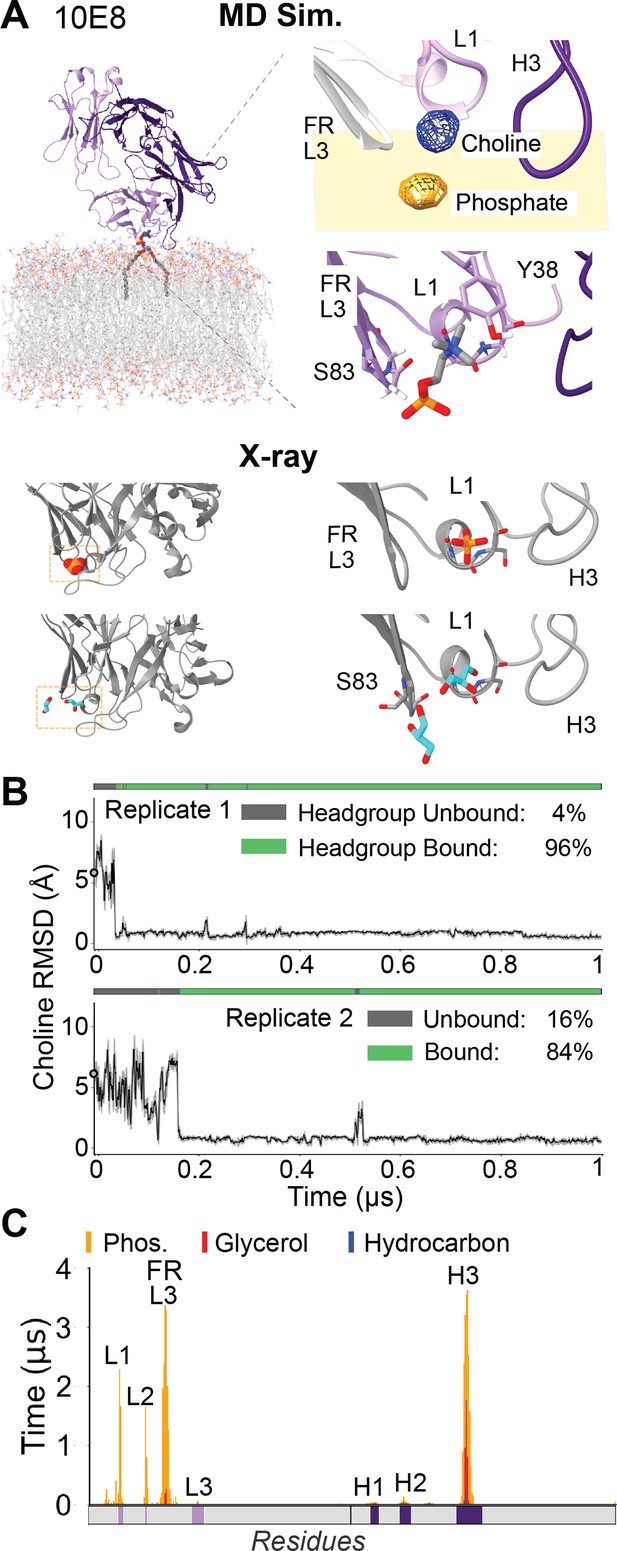
10E8 bivalent lipid headgroup interaction and bilayer insertion predicted in MD simulationagrees with proposed crystallographic lipid binding pocket.
(A) Top left, representative frame from MD simulation of lipid interacting with 10E8 Fab. Top right, the MD lipid binding site includes a bivalent choline and phosphate lipid headgroup complex (represented as blue and orange mesh time-averaged positional density in simulations) within a protein surface groove composed of CDR-L1 and FR-L3, respectively. Bottom, positions of phosphates or glycerols modeled at the CDR-L1 and FR-L3 groove site within 10E8 Fab X-ray structures (PDB: 5T85, 5T6L). (B) RMSD of lipid choline position in the MD simulations versus expected CDR-L1 lipid binding site from 10E8 X-ray structures. ‘Bound’ state assigned relative to choline position and phosphate FR-L3 interactions observed in MD. (C) Per-residue interaction profiles for antibody Fab simulations for 10E8 with Fab domain regions making significant contact labeled, including CDRs and light chain framework region 3 (FR-L3).
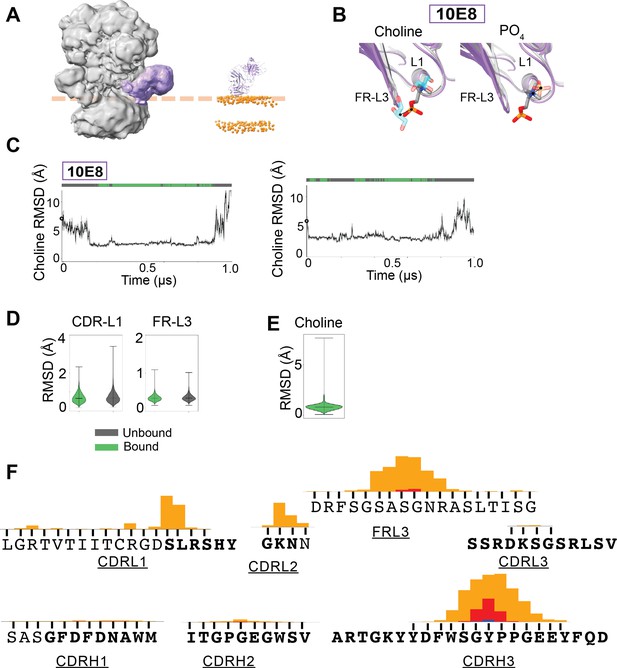
All atom MD replicates for 10E8 detailing molecular interactions in phospholipid binding and membrane association.
(A) Membrane orientation of 3 10E8 Fabs bound to full-length HIV Env trimer (EMD: 25024) inferred from the cryoEM density map compared to the initial docked orientation of 10E8 Fab docked with PPM2.0 used for simulation. (B) In the 10E8 CDR-L1 FR-L3 groove binding site, glycerols (left, partially transparent) and phospholipid headgroups (right, partially transparent) are shown overlain with the bivalent POPC headgroup bound in MD simulations (grey backbone for X-ray, purple for MD). The lipid choline cation forms complementary electrostatic interacts with the CDR-L1 unpaired backbone carbonyls in a helical loop conformation, forming a C-cap style interaction, and overlays with density modeled as free dihydrogen phosphate, lipid phosphoadic, or free glycerol in X-ray structures (Yang et al., 2013). The lipid phosphate accepts hydrogen bonds from the FR-H3. Combination of satisfied polar interaction to both lipid phosphate and choline (<3.5 Å distance) as well as choline RMSD to the relative X-ray structure positions were used to define a phospholipid as ‘bound’ in 10E8 simulations. (C) Two additional replicate all-atom MD simulations of 10E8 bnAb Fab started from varied orientations relative to the membrane, tracking RMSD of MD choline coordinates during the trajectory versus the reference phosphate coordinate from a 10E8 X-ray structure. (D) RMSD per frame distribution of 10E8 CDR-L1 and FR-L3 backbone coordinates versus averaged reference coordinates for 4 µs aggregated simulation time, showing sub-Å RMSF. (E) RMSD of lipid choline headgroup position compared to the heavy atom CDR-L1 binding site position in X-ray structures during the ‘bound’ state for 10E8, showing sub-Å accuracy to experiment. (F) Per-residue interaction profiles for lipid-interacting 10E8 CDR loops and interacting framework regions.
-
Figure 2—figure supplement 1—source data 1
Statistics of each metric used to define geometric macro-substates in global clustering which included aggreagating all simulation time from 10E8, PGZL1, and 4E10 together (Figure 2—figure supplement 1A–C).
Mean and standard deviation for CDRL loop depths, CDRH loops depths and angles of approach and rotation extracted from each substate are reported.
- https://cdn.elifesciences.org/articles/90139/elife-90139-fig2-figsupp1-data1-v1.xlsx
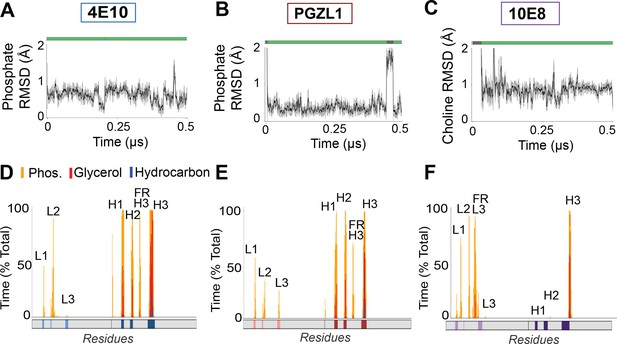
Atomic simulations robustly capture phospholipid binding within predicted sites in realistic HIV-like membranes.
(A) 500 ns simulation of 4E10 bnAb Fab all-atom MD simulations tracking a POPC lipid phosphate binding in a more realistic HIV-like membrane composition. (B) PGZL1 bnAb Fab all-atom MD simulation tracking a PSM lipid phosphate binding events in a more realistic HIV-like membrane composition. (C) 10E8 bnAb Fab all-atom MD simulations tracking choline binding in a more realistic HIV-like membrane composition. (D) Per-residue interaction profiles for lipid-interacting 4E10 CDR loops and framework regions in a realistic HIV-like lipid membrane. Interactions are colored the same as Figure 1E and reported as percent of total time from 500 ns simulations. (E) Per-residue interaction profiles for lipid-interacting PGZL1 CDR loops and framework regions in a realistic HIV-like lipid membrane. (F) Per-residue interaction profiles for lipid-interacting 10E8 CDR loops and framework regions in a realistic HIV-like lipid membrane.
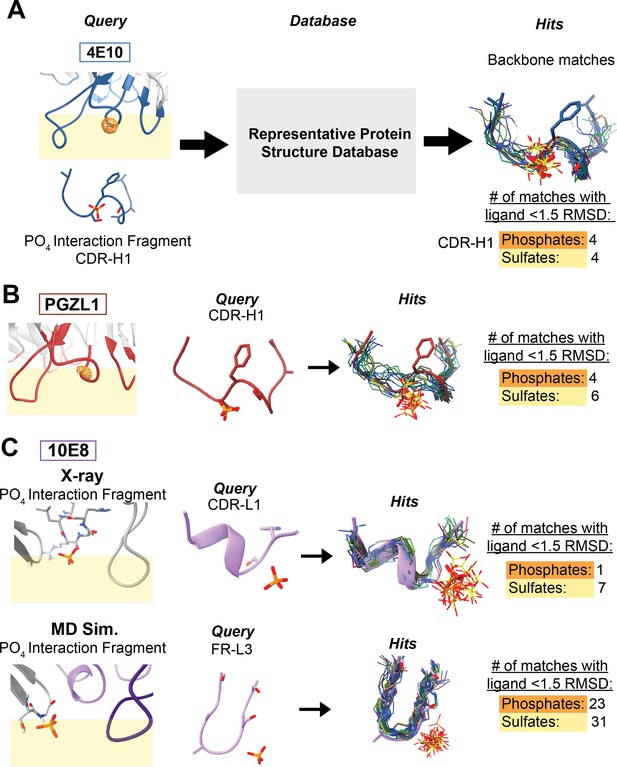
Structural database mining shows bnAb CDR phosphate sites from MD simulations are native-like and present in the proteome.
(A) Local loop geometry from each bnAb Fab simulation was extracted and queried against a 33,372 member database of experimental protein X-ray structures at<2.5Å resolution and<90% sequence identity previously described in Polizzi and DeGrado, 2020. Those protein structures from the database with loops having high structural similarity to the bnAb Fab loops (<2 backbone atom RMSD) were identified, then further mined for the presence of crystallographic phosphate or phosphoryl groups bound in the loop within a 1.5 Å radius the reference phosphate coordinate in the experimental 4E10 bnAb structure at the CDR H1 loop. The example of the 4E10 CDR-H1 phosphate site is shown, with four matching examples of similar loops of distinct primary sequence binding phosphates analogously to 4E10: three matches from a family of bacterial bis-phosphate aldolase enzymes with organophosphate ligands, and one from human chymotrypsin C (PO4 ligand). Many sulfate and sulfo-group containing ligands were found as well, including the ‘positive control’ of similar PGZL1 with a SO4 bound at CDRH1 (PDB:6O3K). (B) Examples of loops matching PGZL1 CDR-H1 with bound phosphate/phosphoryl groups and sulfate/sulfo groups were similar to those for 4E10, in line with these two antibodies’ loops’ structural similarity. (C) Given the shift of lipid phosphate site in the previous X-ray structures versus our MD simulations, we queried both the CDR-L1 loop in the X-ray structure and the FR-L2 separately to interrogate the biological relevance of each putative phosphate binding site through its prevalence in natural proteins of distinct structure. The CDR-L1 phosphate binding site (top) has a geometry which proved to be infrequent in the PDB, matching only 1 other instance. In contrast, the FR-L2 phosphate site (bottom) discovered using MD was far more common in the proteome, found in different protein families including ligase, lyase, and oxidoreductase enzymes with organic phosphoester-containing ligands.
-
Figure 2—figure supplement 3—source data 1
Bioinformatics descriptions for ligand bound structural matches and benchmarking Loop Conformations.
Lipid interacting loop fragments from MD simulations or crystal structures were queried against structural database and hits were filtered to include ligands within 1.5 angstroms from experimental or simulation predicted phosphate or choline interactions. Relevant information for hits from each queried loop fragment includes PDB code, structural entry name in The Protein Data Bank, bound ligand ID, organism from which the protein structure is isolated, and functional classification. Number of fragments found based on a range of RMSD cutoffs for structural searches with phosphate binding fragments of MPER bnAbs as input query structures. Intermediate stringency is found at a 2Å RMSD cutoff. Identified hits with a 2Å RMSD cutoff using MPER bnAb phosphate binding fragments as queries compared to other structural queries with similar length but distinct topologies.
- https://cdn.elifesciences.org/articles/90139/elife-90139-fig2-figsupp3-data1-v1.xls
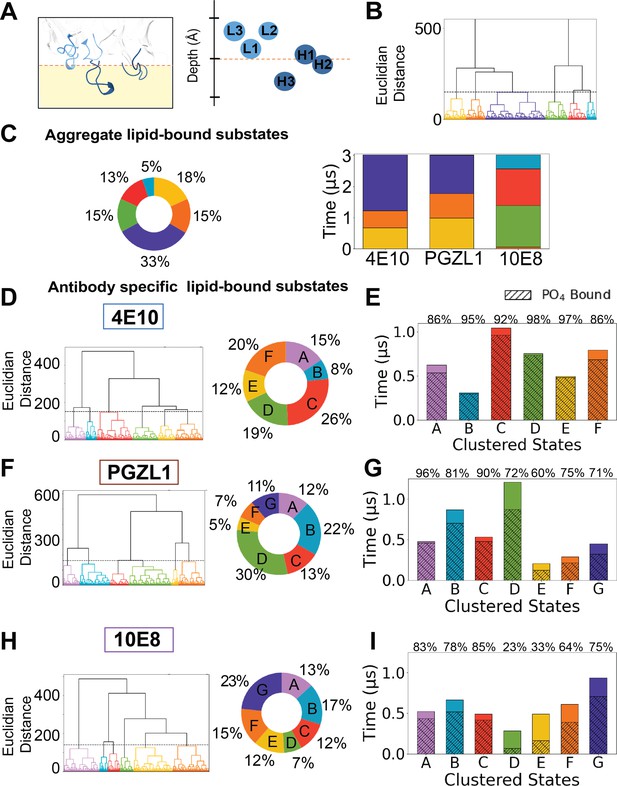
Aggregated and independent geometric clustering of bnAb surface-bound conformations.
(A) Geometric representation of CDR loop depths in membrane where each loop is represented by the center of mass of loop residues and the top of the membrane is represented by average Z-position of lipid phosphates in upper bilayer leaflet. (B) Hierarchical clustering tree of 9 µs simulation time aggregated from trajectories initiated from unique starting membrane-bound conformations (0 degrees, –15 degrees, 15 degrees initialization relative to the docked pose) for the three antibodies 4E10, PGZL1, and 10E8 structurally clustered together aggregated for their protein-membrane interaction geometries. The features defining the substates, that is the feature vector used for structural clustering, include CDR loop depths as well as the Fab global approach and rotational angles (see methods). (C) Classification of sub-states after aggregate clustering of each bnAb Fab’s 3 µs simulation time together, clusters and percent of frames each represented by distinct colors (left). Frames from 4E10 and PGZL1 simulations cluster together, while 10E8 frames are mostly structurally divergent (right). (D–F) Left, geometric clustering and distribution of time each Fab spent in each lipid-bound micro-state across aggregate 4 µs MD simulation time defined from independent clustering. Right, within each lipid-bound substate, the percentage of time with a phosphate group or headgroup defined as bound to the observed interaction site is listed and denoted as hatched shading on each bar graph.
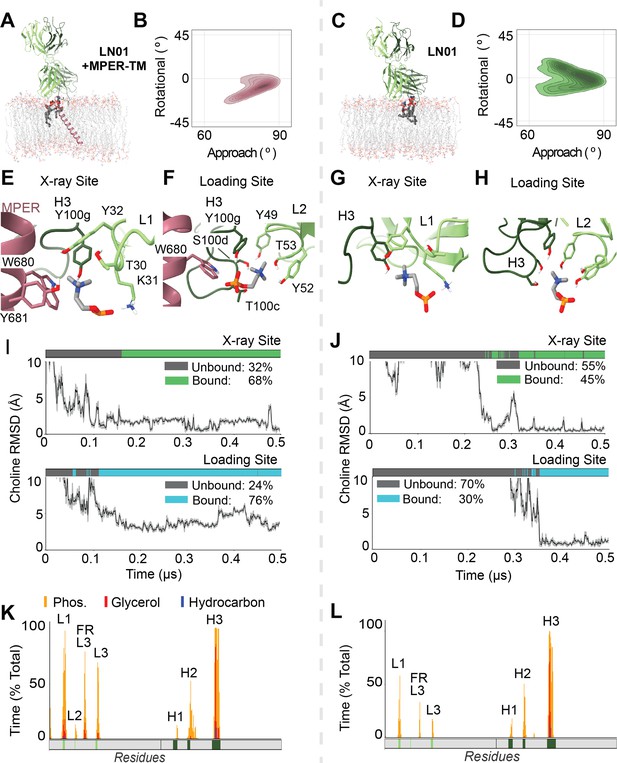
Atomistic simulations of apo and antigen-bound LN01 characterizing paratope-phospholipid complexes with and without epitope and shifted membrane-bound conformation.
(A) Representative frame from MD simulation LN01 Fab bound to MPER-TM showing the stable ternary paratope-epitope-membrane complex; bound phospholipids shown. (B) Frequency of Fab’s characteristic surface-bound geometry by global domain rotation and approach angles in MD simulations for LN01 bound to MPER-TM, plotted by kernel density estimation as contour. (C) Representative frame from MD simulation of phospholipids complexed with LN01 Fab alone. (D) Frequency of geometries sampled for apo membrane-bound LN01. (E) Phospholipid headgroup interaction formed ab initio in LN01 +MPER-TM simulations. Aromatic cation-pi cage motifs coordinate choline while the phosphate is coordinating by Lys31 matching the X-ray site binding pose. (F) The additional distal ‘Loading’ phospholipid site predicted in LN01simulations, with a similar cation-pi cage motif and hydrogen bonds interactions stabilizing the PC headgroup. (G) Atomic interactions at the X-ray site in apo LN01 simulations. (H) Interactions at the loading site in apo LN01 simulations. (I) Lipid headgroup binding in representative simulation of LN01 +MPER-TM (n=4 total). Top, X-ray binding site occupancy (green) and phospholipid choline RMSD in a representative trajectory versus experimental position. Bottom, loading site occupancy (cyan) and choline RMSD versus average headgroup bound position. (J) Lipid headgroup binding for representative apo LN01 trajectory (n=4 total). Site occupancy and RMSD versus predicted binding position for X-ray site (top) and loading site (bottom). (K) Per-residue interaction profile for MPER-TM-bound LN01 aggregated 1.5 µs from 3 simulations. CDR loops are mapped in solid color blocks below each profile. Fab domain regions making significant contact are labeled, including CDRs and light chain framework region 3 (FR-L3). (L) Per-residue interaction profile for apo LN01.
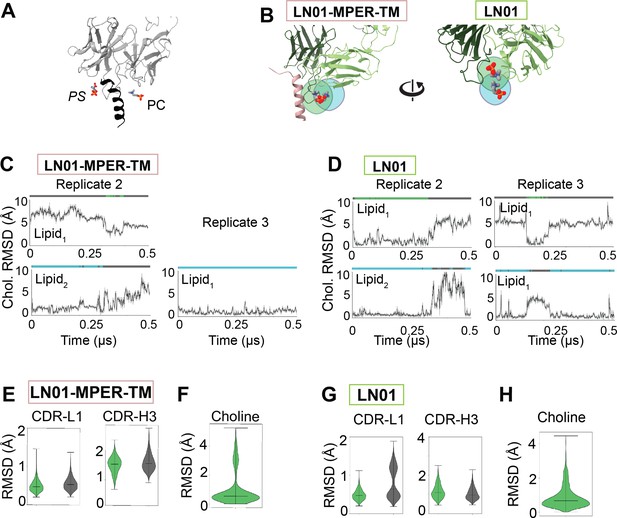
All atom MD replicates LN01-MPER-TM and LN01 with phosphate group interactions in X-ray and loading sites.
(A) Composite of LN01-TM X-ray structures with phosphocholine (PC) bound within the groove of TM and CDR-H3-L1-L2 site (PDB: 6SND, 6SNE, 6SNC) and phosphatidyl-l-serine (PS) bound in a solvent exposed CDR-H1-H2-H3 site (PDB:6SND). (B) Fabs (green cartoon) depict the different lipid binding site locations predicted within MD simulations, including a site analogous to the X-ray PC site (green outline) and the new Loading site (blue outline) positions relative to each other in TM bound and apo systems. (C) Additional replicates of all-atom MD simulations for LN01 +TM showing reproducible lipid phosphate binding event in both the X-ray PC site (top, green) and the loading site (bottom, cyan). No lipid phosphate interaction in X-ray PC site was captured in Replicate 3. (D) Additional replicates of all-atom MD simulations for LN0 showing reproducible lipid phosphate binding event in both the X-ray PC site (top, green) and the loading site (bottom, cyan). A lipid exchange event between X-ray and loading sites is captured in Replicate 3. (E) RMSD per frame distribution of LN01 CDR-L1 and CDR-H3 backbone coordinates versus averaged reference coordinates for LN01 +MPER TM aggregated simulation time. (F) RMSD of lipid choline headgroup position compared to X-ray structure positions during bound time for LN01 +MPER TM systems. (G) RMSD per frame distribution of LN01 CDR-L1 and CDR-H3 backbone coordinates versus averaged reference coordinates for LN01 aggregated simulation time. (H) RMSD of lipid choline headgroup position compared to X-ray structure positions during bound time for LN01 systems.
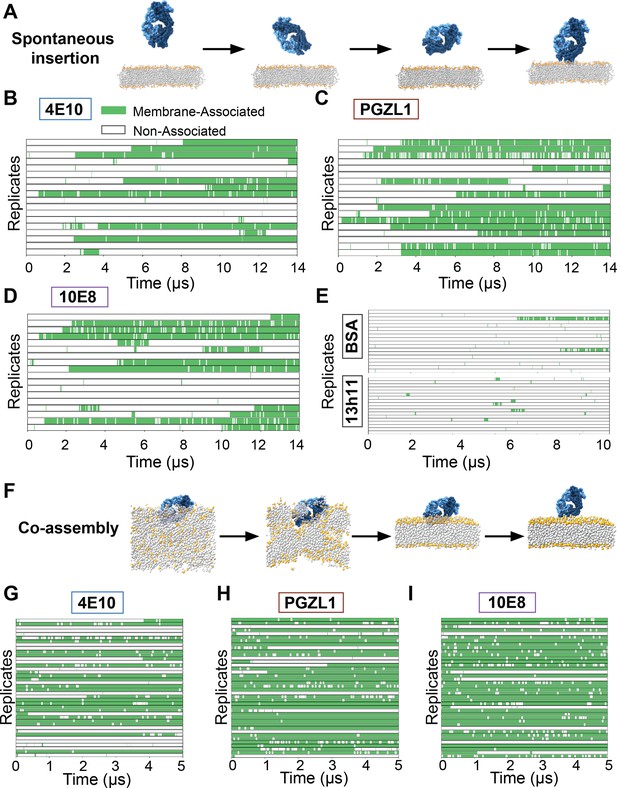
Unbiased spontaneous membrane insertion events and semi-biased dissociation events in coarse grain MD simulations.
(A) Snapshots of spontaneous insertion event from a Martini model coarse-grain simulation of a 4E10 Fab. The Fab begins in explicit water solvent 0.5–2 nm above a lipid bilayer, freely diffusing and tumbling in bulk solvent, often resulting in a temporary or permanent insertion event (right). (B–D) 18 replicates of coarse grain Fab systems (4E10, PGZL1, 10E8, respectively), initialized with slightly different Fab orientations relative to lipid bilayer. Frames with Fab contacting the membrane are in green and frames with Fab in water (non-associated) are in white for replicate trajectories of 14 µs each. (E) 18 replicates of coarse grain BSA (top) or 13h11 (bottom) with different starting orientations relative to the lipid bilayer. Membrane contact (green) or diffusion in water (white) shown over 10 µs time. (F) Snapshots describing a co-assembling membrane pipeline with 4E10 Fab. An Fab is centered in a box with various rotational orientations in space, explicit water, and lipids randomly arranged within a subset of the box (left). By 30ns, the membrane is fully formed (middle). Fab molecules result in a pre-docked membrane bound conformation and sample a permanent insertion event, intermittent membrane association, or dissociation depending on how the Fab contacts with the membrane (right). (G–I) 40 replicates of 5 µs simulations for coarse grain co-assembling systems (4E10, PGZL1, 10E8, respectively), each with slightly different Fab initial orientations relative to lipid bilayer. Membrane contact is classified as above.
-
Figure 4—source data 1
Spontaneously inserted coarse grain membrane contact events for bnAb Fabs.
Description of 14 µs coarse grain simulations for spontaneously inserting 4E10, PGZl1, and 10E8 antibody Fabs. Description of 10 µs coarse grain simulations for negative control systems using BSA and 13h11 Fab are included. Membrane association time is reported for each replicate that was initiated from various orientations relative to the membrane plane, along with total membrane contact time for each Fab system. Average contact time and standard deviation from total membrane contact time is reported. Average contact time and standard deviation per each association event in each antibody systems is also reported.
- https://cdn.elifesciences.org/articles/90139/elife-90139-fig4-data1-v1.xlsx
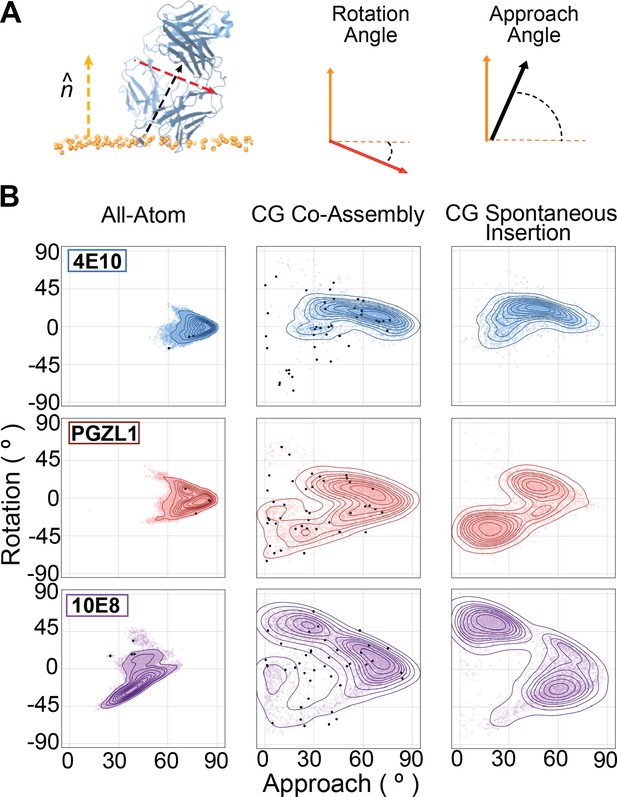
Membrane surface-bound bnAb conformations sampled across multiscale simulations.
(A) Graphic of angles defined to describe Fab geometries relative to the normal vector at the membrane’s upper leaflet lipid at the phosphate plane in simulation frames (orange arrow). The canonical ‘approach angle’ defines the long axis of the Fab domain (i.e. the central pseudo-symmetry axis) and membrane normal vector (black arrow). A second ‘rotational angle’ is defines the global domain rotation about the Fab pseudo-symmetry axis relative to the membrane normal vector, based on the short axis traversing the light and heavy chains, which is nearly orthogonal to the Fab’s central axis (red arrow). (B) Frequency plots of rotation and approach angles from frames of membrane-bound Fabs in MD simulations for 4E10 (blue, top row), PGZL1 (red, middle row), and 10E8 (purple, bottom row). Contour plots depicting frequency maxima for angle pairs sampled are by kernel density estimation. Left column, membrane interaction angles sampled from all-atom simulations with Fabs pre-docked using OPM PPM server prediction. Middle column, geometries from coarse-grain membrane co-assembly simulations. Right column, geometries from unbiased spontaneous insertion coarse-grain simulations. Black dots denote the initial Fab-membrane geometries of starting states for replicate trajectories for each antibody initiated in the lipid bilayer.
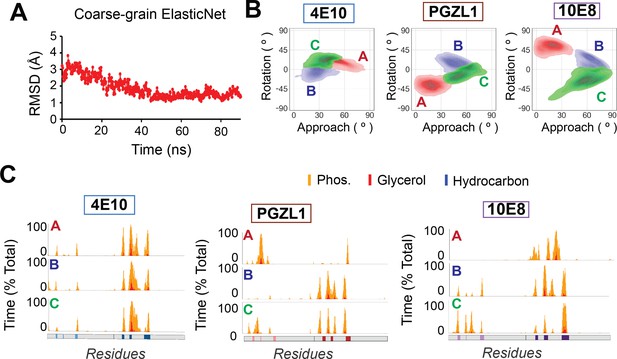
Stability and membrane interactions from coarse-grain expanded sampling.
(A) The backbone RMSD of a 4E10 Fab coarse grained with the Martini force field and restrained with the ElasticNet model maintains. (B) Frequency of membrane interaction angles from coarse grain spontaneous insertion as clustered by geometric substates for 4E10, PGZL1 and 10E8, colored and contoured as in Figure 3B. (C) Interaction profiles for the coarse-grained spontaneously inserted frames that were clustered into substates A-C based on geometric angles. The percent of total time of each coarse grain substate for 4E10 (left), PGZL1 (middle), and 10E8 (right).
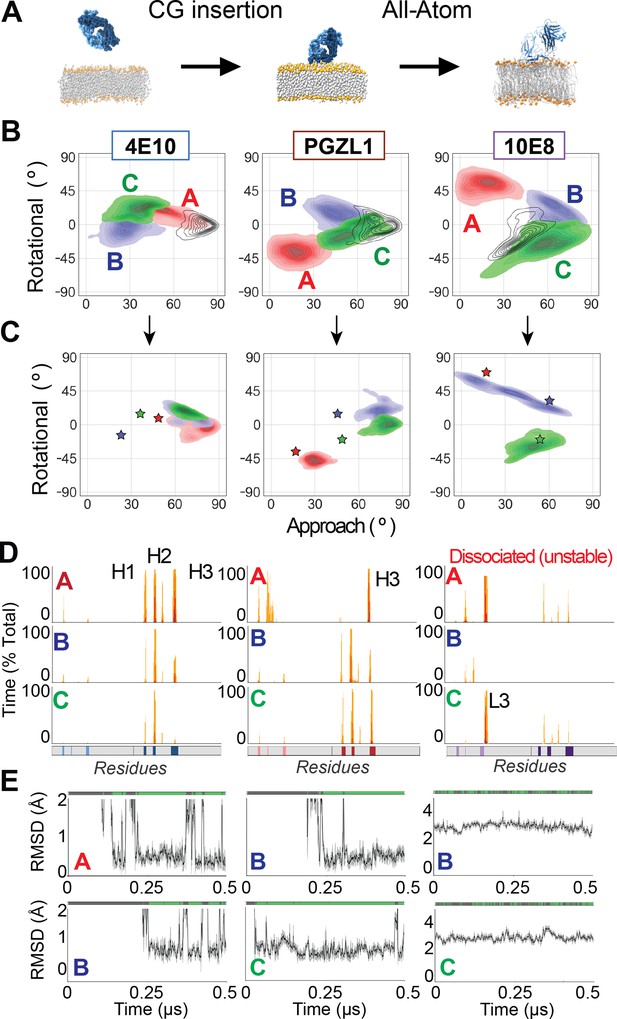
Back-mapping CG-membrane-bound geometries to all-atom simulations allows integrative ab initio modeling of the full bnAb insertion process.
(A) Representative frames from membrane-bound 4E10 Fab coarse-grained simulations were back-mapped to all-atom representation to assess the stability and plausibility of those membrane-bound conformations. This coarse-grained-to-all-atom (CG-to-AA) reversion was applied for all medoid frames of interest from each antibody system and used to initiate half-microsecond unbiased all-atom dynamics simulations. (B) Frequency of membrane interaction angles from coarse grain spontaneous insertion as clustered by geometric substates for 4E10, PGZL1, and 10E8, colored and contoured as in Figure 3B. Corresponding primary all-atom simulations is overlaid (unfilled, black contour frequency density plot). (C) Conformational geometry sampled upon conversion of CG medoid to an all-atom trajectory. Initial geometry denoted by stars colored matching CG clusters in (B). Frequency and contour plots of conformational angles sampled in stably inserted backmapped all-atom trajectories for 4E10 (left), PGZL1 (middle), and 10E8 (right). (D) Per-residue interaction profiles for antibody Fab simulations for 4E10 (left), PGZL1 (middle), 10E8 (right) representing each backmapped atomic trajectory, showing CDR-mediated conformations of differing depths and geometries. (E) Phospholipid headgroup binding and RMSD plots of closest lipid at respective experimentally determined CDR sites for 4E10 (left), PGZL1 (middle), and 10E8 (right), plotted as in Figure 1C or Figure 2B. 4E10 Cluster A is reported in supplement, PGZL1 Cluster A is not reported because no lipid binding was detected, and 10E8 Cluster A is reported in supplement as an artifact.
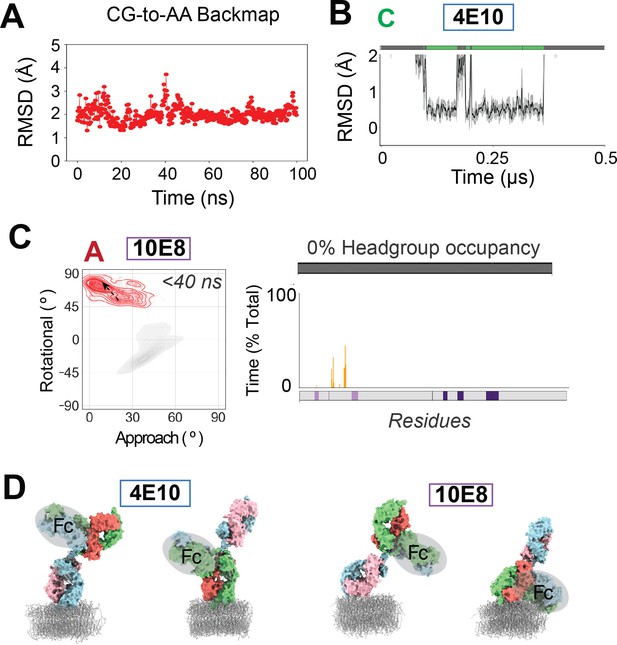
Coarse grain to all atom backmapping validation.
(A) The backbone RMSD of a 4E10 Fab backmapped from coarse grain to atomistic representation remains stable in an all-atom equilibration simulation. (B) The backmapped atomic State C for 4E10 demonstrated lipid headgroup binding in the expected CDR-H1 site. (C) Backmapped state A for 10E8 proved an artifact after dissociating within 40 ns of atomic simulation. We observed alternative geometry of Fab domain by global orientation angles sampled (red contour) compared to our previous atomic simulations (grey contour, from Figure 2), no lipid headgroup binding (right, top), and a distinct protein-lipid interaction profile during the contact time with sparse surface interactions with lipids (right, bottom). (D) Potential for bivalent Fab domain insertion for the full length IgG of 4E10 (left) based on the membrane-bound conformation, built by structural alignment to a full-length IgG model (PDB: 1HZH). Simultaneous Fab contact from both IgG arms appears improbable based on strain and rotational rearrangements that would be imposed to the IgG hinge region to accommodate such a pose. Frames extracted from 10E8 simulations (right) where a full-length IgG model (PDB: 1HZH) is superposed to membrane-bound Fab imply unlikely bivalent interactions. Representative frames are from two distinct time points from 0 degree tilt replicates where phospholipids were bound for both 4E10 and 10E8, representing at least two distinct macroscopic substates differing in global light chain and heavy chain orientation towards the membrane between the two antibodies.
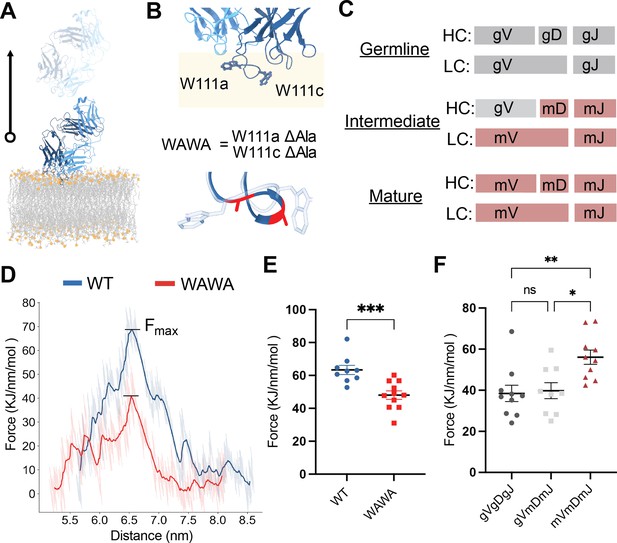
Biased antibody-membrane pulling dissociation simulations approximate Fab-membrane interaction strength for bnAb Fab variants of known lipid binding affinity or neutralization potency.
(A) Schematic of pulling method, a bnAbs Fabs associated with the lipid bilayers is subject to an applied upward dissociation force to the Fab domain center of mass at constant velocity to measure the force required to dissociate the Fab from the bilayer. (B) The Trp100a-Trp100c motif residues in 4E10 CDR-H3 loop of expected to be lipid-embedded in the bilayer (beige). The double alanine mutant ‘WAWA’ 4E10 variant has experimentally determined significantly reduced affinity to lipid bilayers and lower neutralization potency, due to lack of Trp membrane insertion. (C) Previously experimentally characterized (Huang et al., 2012) PGZL1 germline-reverted variants, shown as chimera of germline versus matured gene segments, used to approximate antibody properties along its maturation trajectory. (D) Average force versus distance plots and rupture force (Fmax) calculation for one replicate of pulling wild type 4E10 (WT, blue) and 4E10 WAWA (red) to bias Fab dissociation from the bilayer. (E) Distribution of rupture forces required for dissociation for different membrane-bound starting conformations for 4E10 (n=9, blue) and 4E10 WAWA (n=11, red), with starting conformations drawn from previous unbiased all-atom simulation ensembles at rest. Outliers as dots (F) Distributions of rupture forces (n=10) required for membrane dissociation of PGZL1 inferred variants along the maturation pathway, for germline (dark grey, gVgDgJ), intermediate (light grey, gVmDmJ), and mature (maroon, mVmDmJ) PGZL1. A one-tailed two-sample t-test was performed to analyze statistical significance of rupture forces between two antibodies (*=p < 0.05; **=p < 0.005; ***=p < 0.001).
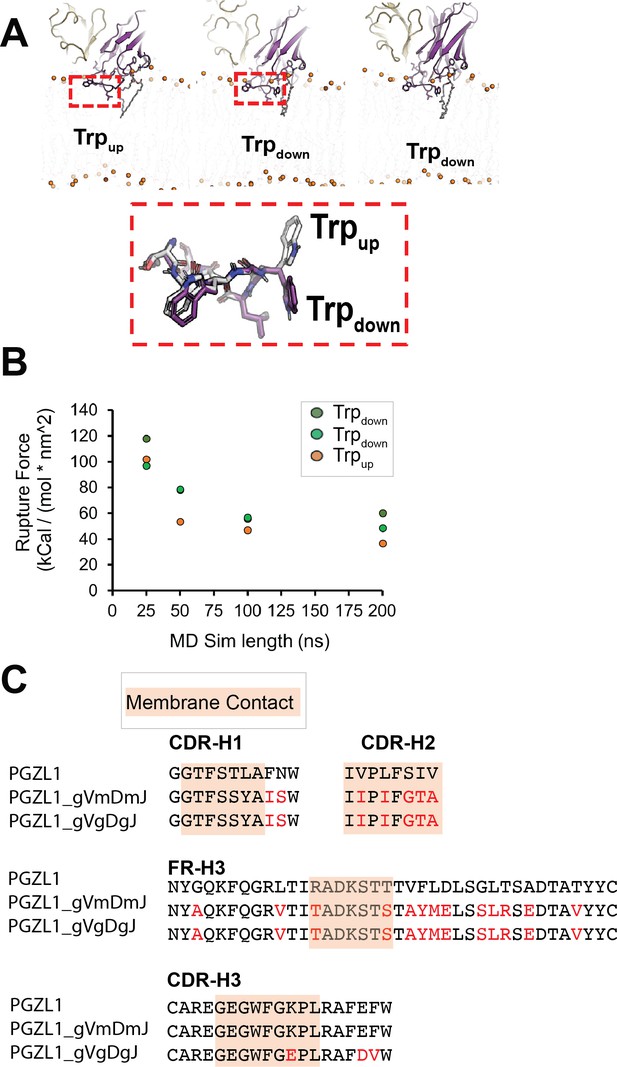
Constant velocity pulling simulations are sensitive to dynamics and sequence variation.
(A) Rotameric states of Trp100a and Trp100b residues in the CDR-H3 loop of 4E10. Distinct starting conformations of TrpUp and TrpDown models used in pulling simulations are shown. (B) Max force for each wildtype 4E10 biased membrane-dissociation replicate simulation is plotted against the time of pulling simulation across fixed distance from the membrane, thus testing different constant pulling velocities. A clear difference for rupture force is detectable for 4E10 replicates initiated from the TrpUp versus TrpDown conformation, most noticeable at 50, 100, and 200 ns. Pulling trajectories are nearly identical for replicates initiated from poses adopting two TrpDown state (green) at 50 and 100 ns. Max force values roughly plateau between 100 and 200 ns. (C) A sequence alignment for germline, intermediate, and mature PGZL1 variants of regions that contact the membrane during primary atomistic simulations with mature PGZL1 (CDR-H1,H2,H3 and FR-H3).
Videos
De novo predicted phosphate binding in atomistic 4E10 Fab MD simulation Atomistic simulation of 4E10 Fab (heavy chain: dark blue, light chain: light blue) initially docked to the membrane using OPM PPM server prediction.
Lipids and cholesterol are shown as grey sticks with phosphates from top and bottom leaflets shown as orange spheres. The binding phosphate (large orange sphere) from a POPC lipid is initially more than 10 Å away from CDR-H1 loop and finds interaction with CDR-H1 residues in first 200 ns of simulation and maintains for remainder of 1 µs.
Phosphate binding and replacement event in PGZL1 bnAb Fab all-atom simulation Atomistic simulation of PGZL1 Fab (heavy chain: salmon, light chain: light pink) initially docked to the membrane using OPM PPM server prediction.
Lipids and cholesterol are shown as grey sticks with phosphates from top and bottom leaflets shown as orange spheres. The first interacting phosphate from a POPC lipid (large orange sphere) is initially more than 6 Å away from CDR-H1 loop and binds in CDR-H1 loop for first 500 ns of simulation. A second phosphate from a POPA lipid (pink sphere) replaces initial interaction around 550 ns and maintains the CDR-H1 interactions for the rest of the microsecond simulation.
Unbiased spontaneous membrane insertion event in coarse grain 4E10 Fab MD simulation Coarse grain 4E10 Fab (blue) is initialized from 2 nm above an assembled membrane (grey lipid tails and cholesterol and orange phosphates).
Random diffusion and tumbling in explicit water is observed before initial membrane contact is made. Membrane association is followed by reorganization of the Fab-membrane conformation for the remainder of the 14 µs simulation.