Reassessing the substrate specificities of the major Staphylococcus aureus peptidoglycan hydrolases lysostaphin and LytM
Figures
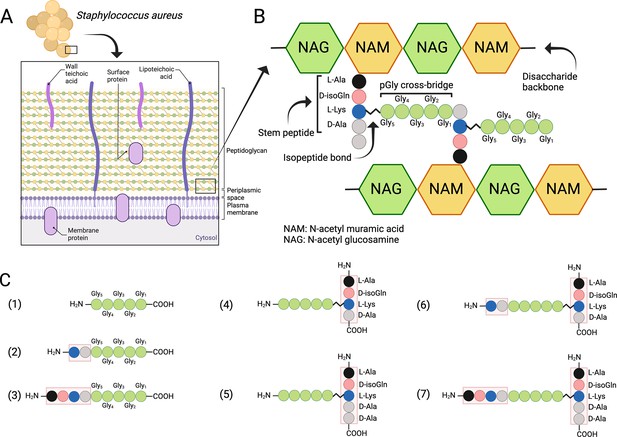
Structure of the cell wall peptidoglycan (PG) in S. aureus.
(A) Schematic overview of the cell wall in S. aureus. (B) Structure of the PG. (C) Schematic presentation of peptides used to study target bond specificity of the enzymes. This figure was created using BioRender.com.
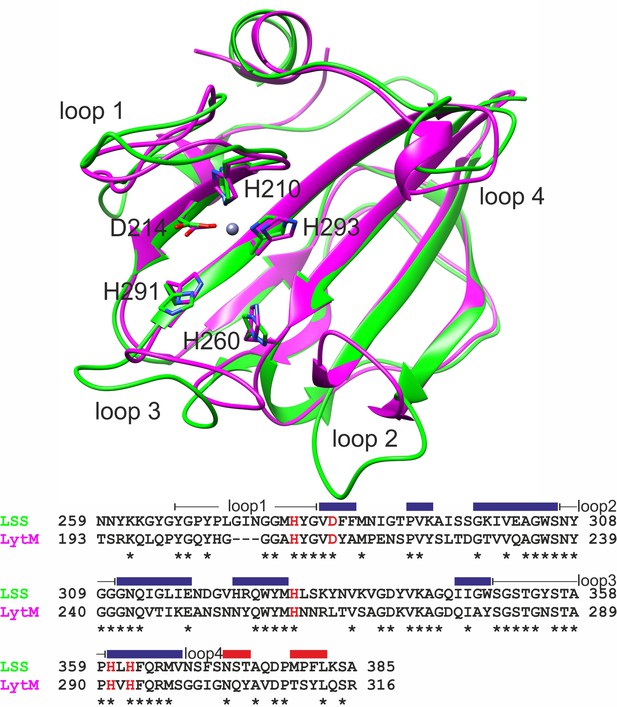
Overlaid structures of the catalytic domains of lysostaphin (LSS) (green, PDB ID 5NMY) and LytM (magenta, PDB ID 2B13) as well as their aligned amino acid sequences.
Sidechains of zinc-coordinating and catalytic residues are shown in sticks and highlighted in red in the sequence alignment. Loops surrounding the catalytic groove are numbered from 1 to 4, and together with secondary structures (β strands in blue and α helices in red) shown for LytM above the sequence.
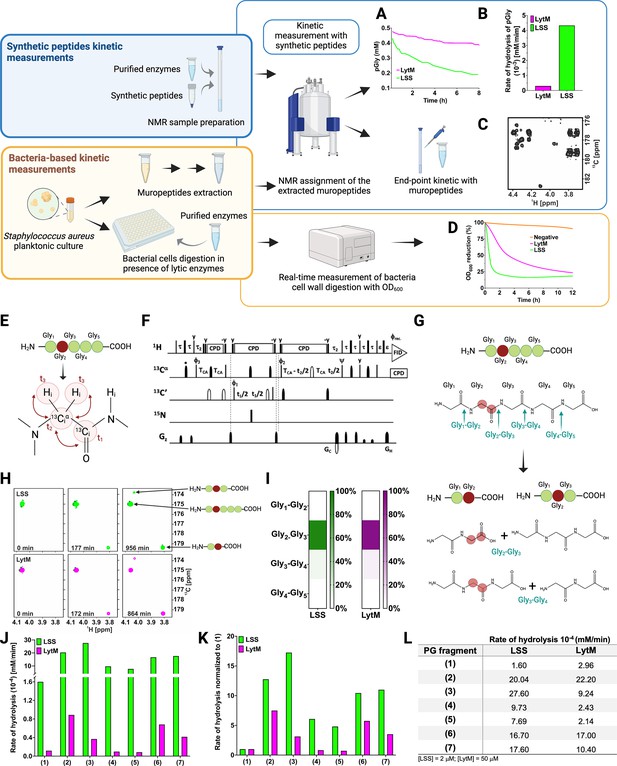
Workflow to study M23 peptidoglycan hydrolase (PGH) substrate specificities.
Panels in the upper left corner, the two main strategies used in the study. Kinetic measurements carried out with peptidoglycan (PG) fragments (synthetic peptides) were supported by bacteria-based kinetic measurements using S. aureus USA300 cells. Panels in the upper right corner, (A) hydrolysis of synthetic pGly (mM) by lysostaphin (LSS) (green) and LytM (magenta) monitored by 1H NMR spectroscopy over time (hr). Both enzymes were used at the concentration of 50 μM. (B) Rate of hydrolysis (mM/min) of pGly derived from A in the first 60 min of the reaction for LSS and LytM. (C) 13C-HMBC NMR spectrum showing the end-point kinetic of LSS-treated muropeptides extracted from S. aureus USA300 cells. (D) Turbidity assay using S. aureus USA300 cells in the presence of LSS and LytM at a concentration of 3 μM. The cell lysis is expressed as percentage reduction of the bacteria suspension optical density at 600 nm over time (hr). (E) Pentaglycine hydrolysis by LSS and LytM was studied by using a Gly213C-labelled substrate. (F) NMR pulse sequence for the acquisition of glycine Hα-detection optimised two-dimensional (2D) HA(CA)CO spectra, showing correlations between 1Hα and 13CO atoms. (G) With a label the otherwise identical products of the two hydrolysis reactions G2-G3, G3-G4 can now be differentiated. (H) The order of appearance of the peaks of the labelled products as a function of time. The labelled glycine has a different 13CO shift when as G2 in triglycine or G2 in diglycine. (I) Heatmap summarising the bond preferences for the enzymes in the pGly. Hydrolysis of peptides 1–7 by LSS (green) and LytM (magenta). (J) Initial rates of substrate hydrolysis (mM/min) of LSS and LytM at 2 μM concentration and (K) the same rates normalised to that of pGly. (L) Absolute values of rates of hydrolysis. For PG fragments 2 and 3, two independent measurements were performed to test and accredit the reproducibility of the method (see Materials and methods). This figure was created using BioRender.com.
-
Figure 2—source data 1
Raw Excel data for Figure 2D.
- https://cdn.elifesciences.org/articles/93673/elife-93673-fig2-data1-v1.xlsx
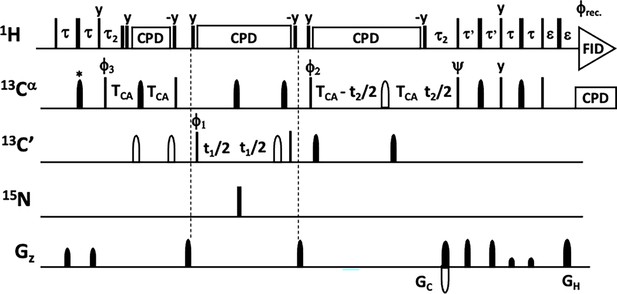
Glycine-optimised HCACO experiment for correlating 1Ha, 13Ca, 13CO resonances.
Narrow and wide filled bars on 1H and 15N channels correspond to rectangular 90° and 180° pulses, respectively, applied with phase x unless otherwise stated. All 13C pulses are band-selective shaped pulses, denoted by filled narrow bars (90°) and filled and unfilled half ellipsoids (180°). Pulses denoted with unfilled bars are applied on-resonance. The 1H, 15N, 13CO, and 13Cα carrier positions are 4.7 (water), 118 (centre of 15N spectral region), 174 ppm (centre of 13CO spectral region), and 54 ppm (centre of 13Cα spectral region). The 13C carrier is initially set to the middle of 13Cα region (45 ppm) and shifted to 13CO region (174 ppm) prior to 90° 13CO pulse Φ1. All band-selective 90° and 180° pulses for 13Cα (45 ppm) and 13CO (174 ppm) have the shape of Q5 and Q3 (Emsley and Bodenhausen, 1992) and duration of 240.0 μs and 192.0 μs at 800 MHz, respectively. The Waltz-65 sequence (Zhou et al., 2007) with strength of 4.17 kHz was employed to decouple 1H spins. The GARP (Shaka et al., 1985; Shaka and Keeler, 1987) with field strength of 4.55 kHz was used to decouple 13C during acquisition. Delay durations: τ=1/(4JHC)~1.7 ms; τ’=0.85 ms; τ2=1.7 ms (optimised for glycine residues) or τ2=2.2–2.6 ms (for observing both glycine and non-glycine residues); ε=duration of GH+field recovery ~1.2 ms; 2TCA = 1/(2JCαCO)~9.5 ms. Maximum t2 is restrained t2,max<2.0*(TCA - τ2). Frequency discrimination in 13CO dimension is obtained using the States-TPPI protocol (Marion et al., 1989) applied to Φ1, whereas the quadrature detection in 13Cα dimension is obtained using the sensitivity-enhanced gradient selection (Kay et al., 1992; Schleucher et al., 1994). The echo and anti-echo signals in 13Cα dimension are collected separately by inverting the sign of the GC gradient pulse together with the inversion of ψ, respectively. Phase cycling: Φ1=x, -x; Φ2=2(x), 2(-x); Φ3=4(x), 4(-x); ψ=x; rec.=x, 2(-x), x, -x, 2(x), -x. Gradient strengths (% of max G/cm) and durations (ms) for the coherence selection: GC = 80.0%, 1.0 ms; GH = 20.1% 1.0 ms.
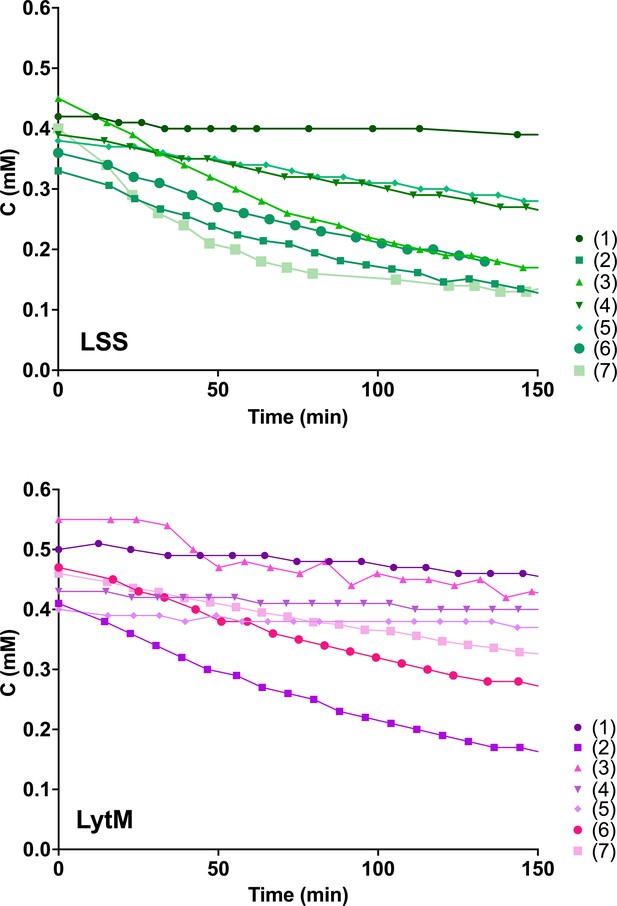
Hydrolysis of peptides 1–7 by lysostaphin (LSS) (green) and LytM (magenta).
Plots of decaying substrate concentration in function of reaction time.
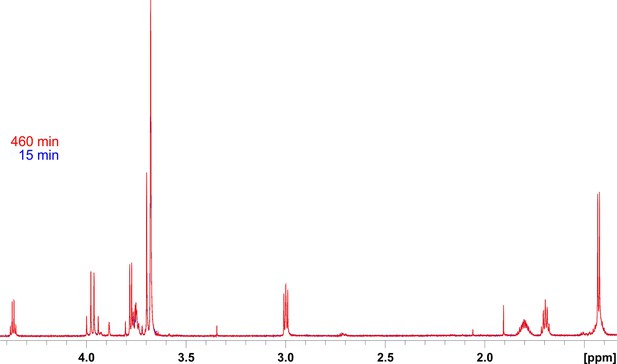
Assessment of the stability of the 800 MHz 1H NMR spectrometer during the relatively long periods of acquisition of kinetics spectra.
Shown are overlaid 1H spectra at the start and end points of the hydrolysis reaction of peptide 14 by lysostaphin (LSS). This peptidoglycan (PG) fragment is not hydrolysed by LSS. Substrate concentration was 0.4 mM and that of LSS 2 μM.
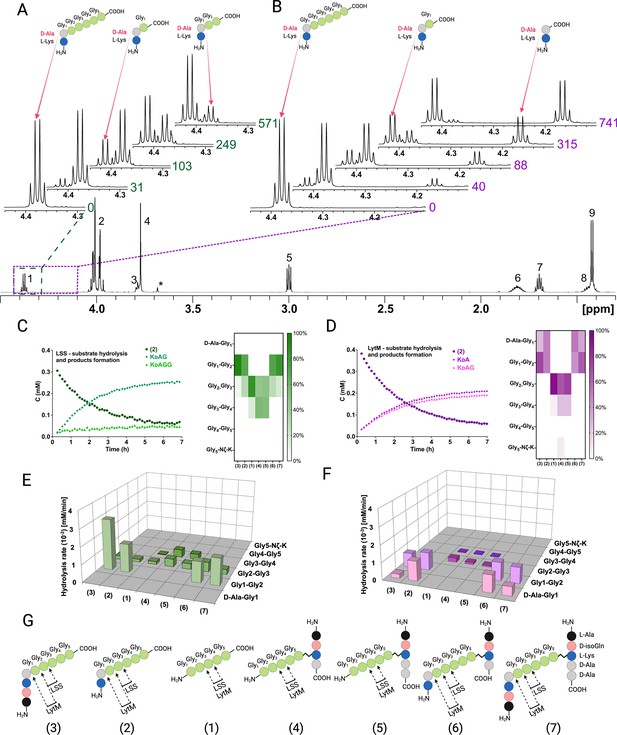
Representative examples of real-time NMR monitoring of substrate hydrolysis.
Quantitative 1H spectra at selected time points in the hydrolysis reactions of peptide 2 by lysostaphin (LSS) (A) and LytM (B). In hydrolysis by LSS peaks of Ala Hα in products KdAG and KdAGG gradually appear as a function of time, whereas in LytM reaction KdA and KdAG are formed. Time points are given in minutes next to the spectra. Peak assignments in the reference spectrum are the following: 1 Ala Hα, 2 Gly1-Gly4 Hα, 3 Lys Hα, 4 Gly5 Hα, 5 Lys Hε, 6 Lys Hβ, 7 Lys Hδ, 8 Lys Hγ, 9 Ala Hβ. Asterisk marks the peak of the buffer. The alanine Hα quartets of substrate (4.373 ppm) and KdAGG (4.365 ppm) partially overlap. (C, D) On the left, concentrations in function of reaction time derived from NMR peak integrals from a typical reaction setup with 0.4 mM peptide and 2 μM LSS or 50 μM LytM. On the right, relative product concentrations at reaction end points for the studied peptidoglycan (PG) fragments. (E, F) Rates of formations of products in hydrolyses by LSS and LytM of the studied PG fragments 1–7. (G) Bonds cleaved by LSS and LytM in different PG fragments. This figure was created using BioRender.com.
-
Figure 3—source data 1
Raw Excel data for Figure 3A.
- https://cdn.elifesciences.org/articles/93673/elife-93673-fig3-data1-v1.xlsx
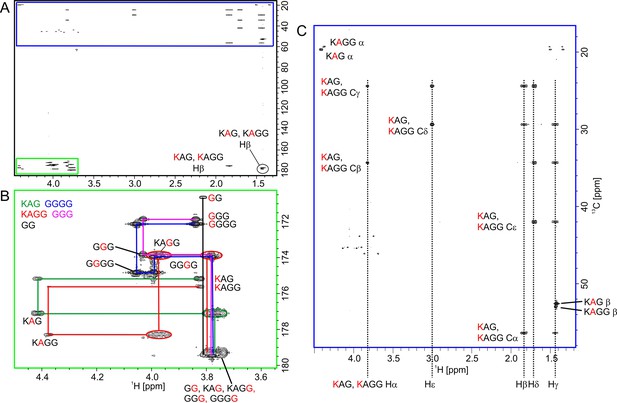
Identification of products in the hydrolysis reaction of peptide 2 by lysostaphin (LSS) with a 13C-HMBC spectrum.
In (A) is shown the full spectrum. Expansions from the boxed regions are shown in (B) corresponding to the Ha-carbonyl carbon region and (C) the aliphatic region. In the former, inter-residual connectivities of the five products are shown with lines of different colours. Diglycine is a secondary product from hydrolysis of tetraglycine.
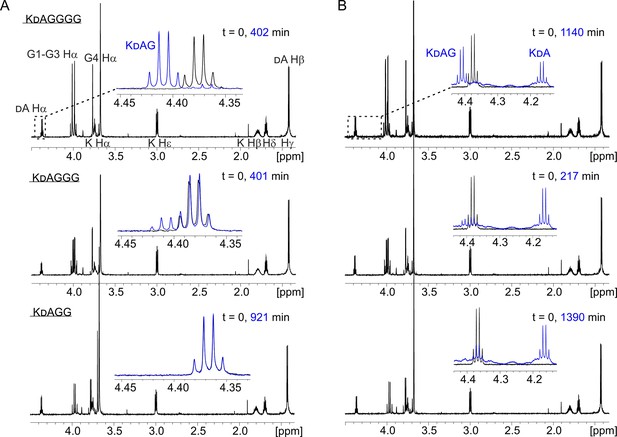
Hydrolysis of peptides KDAGGGG (12), KDAGGG (13), and KDAGG (14) by lysostaphin (LSS) (A) and LytM (B).
In the insets are shown overlays of the boxed dA Ha region of the 1H spectra at two time points of the hydrolysis reactions.
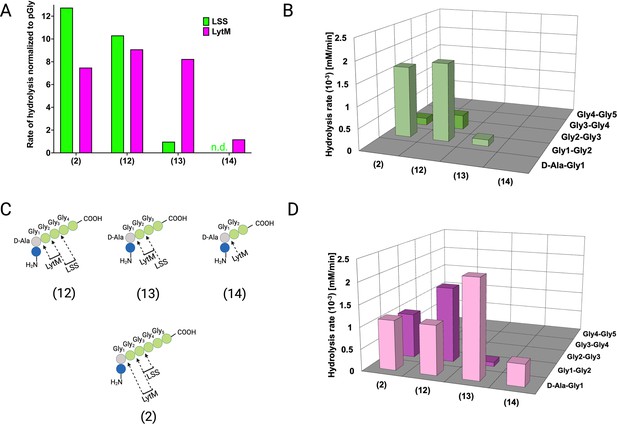
Hydrolysis of peptidoglycan (PG) fragments 12–14 by lysostaphin (LSS) and LytM.
(A) Rate of substrate hydrolysis with respect to pGly. Shown are comparative rates for the substrates 2 and 12–14, having five, four, three, and glycines cross-linked to D-Ala, respectively. (B) Rates of scissile bond hydrolysis in substrates 2, and 12–14 for LSS. (C) Bonds cleaved by LytM and LSS in the PG fragments 2, 12–14. (D) Rates of scissile bond hydrolysis in substrates 2, and 12–14 for LytM. The figure was created using BioRender.com.
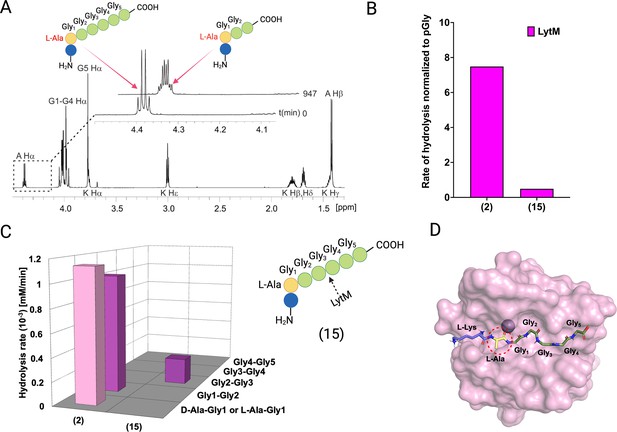
Hydrolysis of peptide KLAGGGGG (15) by LytM.
(A) Contrary to cleavages between Ala-Gly1 and Gly1-Gly2 in KDAGGGGG, LytM now uniquely cleaves between Gly2 and Gly3. The Ha peak at time point 947 min is composed of remaining substrate Ala Ha and Ala Ha from KLAGG. (B) The overall rate of substrate hydrolysis of KLAGGGGG mimics that of pGly and is much slower than hydrolysis of the peptidoglycan (PG) fragment 2. (C) Scissile bond (Gly2-Gly3) is the same as for pGly (1). (D) L-Ala-Gly1 bond hydrolysis is hindered by steric clash of Ala methyl group and loop 3. This figure was created using BioRender.com.
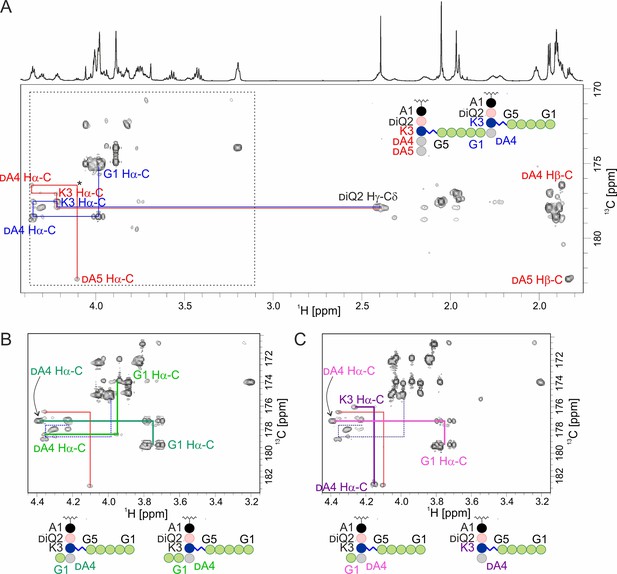
Hydrolysis of muropeptides extracted from S. aureus USA300 sacculus by lysostaphin (LSS) and LytM.
(A) The carbonyl carbon region of a 13C-HMBC spectrum acquired from muropeptides prior to enzyme addition. Inter-residual connectivities and identities are shown for some of the peaks. Colour codes match between the latter and the schematic structure of a peptidoglycan (PG) monomer in the upper right corner. The asterisk marks a peak seen at lower contour levels. (B) The boxed region after reaction with LSS. LSS cleaves the bond between glycines 1 and 2 and a characteristic quartet-like peak pattern of an Ala-linked C-terminal glycine Ha appears (dark green). Based on signal intensities here and those in the 13C-HMBC of reaction products of hydrolysis of 2 by LSS (Figure 1), cleavage between glycines 2 and 3 occurs more seldom (bright green). (C) The boxed region after reaction with LytM. Similar to the LSS-reaction, the G1-G2 bond cleavage product is present (light purple). Additionally, the peaks of a Lys-linked C-terminal alanine appear (dark purple).
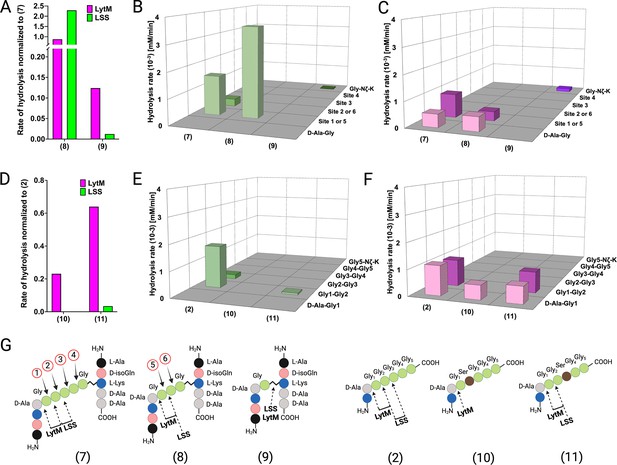
Hydrolysis of peptidoglycan (PG) fragments with a shorter cross-bridge or with serine in cross-bridge.
Rates of substrate hydrolysis of fragments 8 and 9 as compared with 7 by lysostaphin (LSS) (green) and LytM (magenta) (A) and formation of product(s) in hydrolysis by LSS (B) and LytM (C). Rates of substrate hydrolysis of fragments 10 and 11 as compared with 2 (D) and formation of product(s) in hydrolysis by LSS (E) and LytM (F). Depictions of structures of used PG fragments (G). LytM was used at a concentration of 50 μM while LSS was 2 μM. This figure was created using BioRender.com.
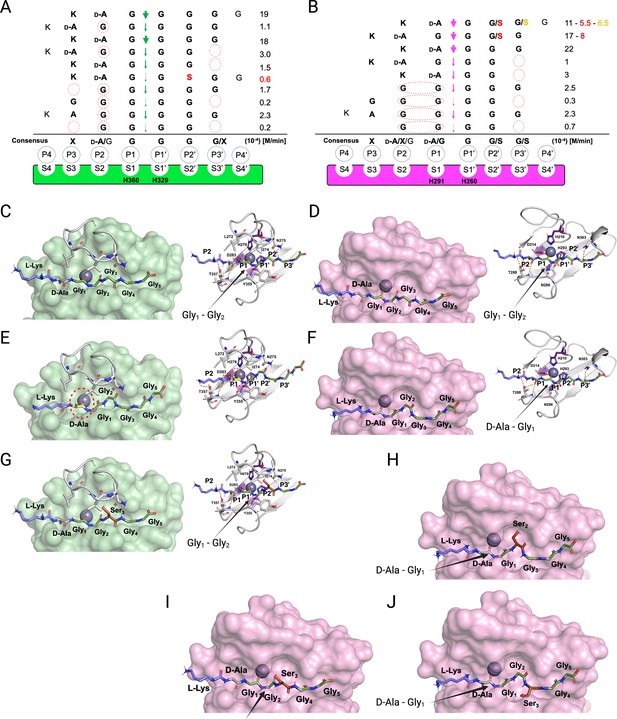
Substrate specificity of lysostaphin (LSS) and LytM.
Schechter and Berger nomenclature is employed to describe the differences in substrate specificity between LSS (A) and LytM (B). Scissile bond in the substrate is between the P1 and P1’ positions, indicated by green (LSS) and purple arrows (LytM), and hence residues towards the N-terminus from the scissile bond are P1-P4, whereas those towards the C-terminus are designated as P1’-P4’. Peptidoglycan (PG) fragments devoid of stem peptide linked to the C-terminal glycine are shown aligned with respect to their cleavage sites together with the rate of hydrolysis of the particular scissile bond. Consensus sequence displays preferable amino acid(s) that are accepted in the specific position (…P2, P1, P1’, P2’…) with respect to the cleavage site. Red circles/ovals indicate missing or less than optimal amino acid accommodation in the particular P site, which translates into reduced catalytic efficiency. Serine substitutions in the glycine bridge and associated rates of hydrolysis are indicated by red and orange colours. (C–F) show the docking results for fragment 2 into the catalytic site of LSS and LytM. (G) Shows docking result of fragment 11 to LSS. (H–J) show the docking results for fragments 10 and 11 into the catalytic site of LytM. LSS and LytM are capable of cleaving the Gly1-Gly2 bond in 2 (C, D). LytM is also able to cleave the D-Ala-Gly1 bond (F), however, in LSS this would result in a steric clash between the D-Ala sidechain and the residues in loop 1 (E). LytM was used at a concentration of 50 μM while LSS was 2 μM. This figure was created using BioRender.com.
Additional files
-
Supplementary file 1
A list of synthetic peptidoglycan (PG) fragments used in this study.
- https://cdn.elifesciences.org/articles/93673/elife-93673-supp1-v1.docx
-
MDAR checklist
- https://cdn.elifesciences.org/articles/93673/elife-93673-mdarchecklist1-v1.docx