Stem-loop and circle-loop TADs generated by directional pairing of boundary elements have distinct physical and regulatory properties
eLife assessment
This valuable work investigates the role of boundary elements in the formation of 3D genome architecture. The authors established a specific model system that allowed them to manipulate boundary elements and examine the resulting genome topology. The work yielded the first demonstration of the existence of stem and circle loops in a genome and confirms a model which had been posited based on extensive prior genetic work, providing insights into how 3D genome topologies affect enhancer–promoter communication. The evidence is solid, although the degree of generalization remains uncertain.
https://doi.org/10.7554/eLife.94114.3.sa0Valuable: Findings that have theoretical or practical implications for a subfield
- Landmark
- Fundamental
- Important
- Valuable
- Useful
Solid: Methods, data and analyses broadly support the claims with only minor weaknesses
- Exceptional
- Compelling
- Convincing
- Solid
- Incomplete
- Inadequate
During the peer-review process the editor and reviewers write an eLife Assessment that summarises the significance of the findings reported in the article (on a scale ranging from landmark to useful) and the strength of the evidence (on a scale ranging from exceptional to inadequate). Learn more about eLife Assessments
Abstract
The chromosomes in multicellular eukaryotes are organized into a series of topologically independent loops called TADs. In flies, TADs are formed by physical interactions between neighboring boundaries. Fly boundaries exhibit distinct partner preferences, and pairing interactions between boundaries are typically orientation-dependent. Pairing can be head-to-tail or head-to-head. The former generates a stem-loop TAD, while the latter gives a circle-loop TAD. The TAD that encompasses the Drosophila even skipped (eve) gene is formed by the head-to-tail pairing of the nhomie and homie boundaries. To explore the relationship between loop topology and the physical and regulatory landscape, we flanked the nhomie boundary region with two attP sites. The attP sites were then used to generate four boundary replacements: λ DNA, nhomie forward (WT orientation), nhomie reverse (opposite of WT orientation), and homie forward (same orientation as WT homie). The nhomie forward replacement restores the WT physical and regulatory landscape: in MicroC experiments, the eve TAD is a ‘volcano’ triangle topped by a plume, and the eve gene and its regulatory elements are sequestered from interactions with neighbors. The λ DNA replacement lacks boundary function: the endpoint of the ‘new’ eve TAD on the nhomie side is ill-defined, and eve stripe enhancers activate a nearby gene, eIF3j. While nhomie reverse and homie forward restore the eve TAD, the topology is a circle-loop, and this changes the local physical and regulatory landscape. In MicroC experiments, the eve TAD interacts with its neighbors, and the plume at the top of the eve triangle peak is converted to a pair of ‘clouds’ of contacts with the next-door TADs. Consistent with the loss of isolation afforded by the stem-loop topology, the eve enhancers weakly activate genes in the neighboring TADs. Conversely, eve function is partially disrupted.
Introduction
Chromosomes in multicellular animals are organized into a series of topologically independent looped domains, called TADs, or topologically associating domains (Cavalheiro et al., 2021; Chetverina et al., 2017; Jerković et al., 2020; Matthews and White, 2019; Rowley and Corces, 2018). The arrangement of TADs in a given chromosomal DNA segment is generally (though not precisely) similar in different tissues and developmental stages, and this is a reflection of the mechanism underlying TAD formation—the endpoints of TADs are determined by a special class of elements called chromatin boundaries or insulators. While boundary-like elements have been identified in a wide range of animals and plants, the properties of this class of DNA elements have been most fully characterized in Drosophila (Cavalheiro et al., 2021; Chetverina et al., 2017). Fly boundaries have one or more large (100–400 bp) nucleosome-free nuclease-hypersensitive sequences that are targets for multiple DNA binding chromosomal architectural proteins. While only a single chromosomal architectural protein, CTCF, has been characterized in mammals, there are several dozen such proteins in flies, and the list is still growing (Heger et al., 2013; Heger and Wiehe, 2014; Schoborg and Labrador, 2010). In addition to subdividing the chromosome into a series of loops, fly boundary elements have insulating activity. When placed between enhancers or silencers and their target promoters, boundaries block regulatory interactions (Bell et al., 2001; Chetverina et al., 2014; Chetverina et al., 2017). This activity provides a mechanism for delimiting units of independent gene activity: genes located between a pair of compatible boundaries are subject to regulatory interactions with enhancers/silencers present in the same chromosomal interval, while they are insulated from the effects of enhancers/silencers located beyond either boundary in adjacent regulatory neighborhoods. It is currently thought that their ability to organize the chromosome into topologically independent loops is important for their insulating activity (Cai and Shen, 2001; Gohl et al., 2011; Muravyova et al., 2001).
Studies dating back to the 1990s have suggested that fly boundaries subdivide the chromosome into loops by physically pairing with each other (Chetverina et al., 2014; Chetverina et al., 2017). In these first experiments, regulatory interactions were observed for transgenes inserted at distant sites in chromosome that were carrying either the gypsy transposon boundary su(Hw) or the bithorax complex (BX-C) boundary Mcp (Muller et al., 1999; Sigrist and Pirrotta, 1997; Vazquez et al., 1993). Further support for the idea that boundaries function by pairing has come from chromatin immunoprecipitation, chromosome conformation capture (CCC), MicroC, and direct imaging experiments (Chen et al., 2018; Li et al., 2011; Vazquez et al., 2006). More recent studies have revealed physical interactions in the CNS, such as those found for su(Hw) and Mcp, which ‘reach over’ multiple intervening TADs, consistent with them playing an important role in cell type-specific gene regulation (Mohana et al., 2023) by bringing distant enhancers and promoters together.
The parameters governing pairing interactions have been defined using insulator bypass, transvection, and boundary competition assays. These studies have shown that fly boundaries are able to pair not only with heterologous boundaries but also with copies of themselves. Moreover, the pairing interactions typically exhibit a number of characteristic features: promiscuity coupled with clear partner preferences, and orientation dependence.
Partner preferences depend upon the chromosomal architectural proteins that interact with each boundary. For example, in the boundary bypass assay, a set of enhancers are placed upstream of two reporters (Cai and Shen, 2001; Kyrchanova et al., 2008a; Muravyova et al., 2001). When multimerized dCTCF sites are placed between the enhancers and the closest reporters, both reporters are insulated from the enhancers. When a second set of multimerized dCTCF sites are placed downstream of the closest reporter, bypass is observed. In this case the closest reporter, which is bracketed by the multimerized dCTCF sites, is still insulated from the enhancers; however, the downstream reporter is activated (Muravyova et al., 2001). Heterologous combinations give a different result: when multimerized dCTCF sites are placed upstream of the closest reporter and multimerized Zw5 sites are placed downstream, no bypass is observed. Endogenous fly boundaries also show partner preferences in bypass assays and in boundary competition experiments (Gohl et al., 2011; Kyrchanova et al., 2011; Kyrchanova et al., 2008b). On the other hand, while boundaries have partner preferences, they are also promiscuous in their ability to establish functional interactions with other boundaries. For example, the Fab-8 insulator can partner with scs’ from the Drosophila heat shock locus (Gohl et al., 2011).
In addition, to partner preferences, pairing interactions between endogenous fly boundaries are, with a few exceptions, orientation-dependent. Self-pairing interactions are head-to-head. This seems to be a common feature of fly boundaries and has been observed for scs, scs’, iA2, wari, Mcp, Fab-8, AB-I, homie, and nhomie (Fujioka et al., 2016; Kyrchanova et al., 2008a). In contrast, pairing interactions between heterologous boundaries can be head-to-head or head-to-tail. The two boundaries bracketing the even-skipped (eve) locus, homie and nhomie, pair with each other head-to-tail, while boundaries in the Abdominal-B (Abd-B) region of the BX-C usually pair with their neighbors head-to-head. The topology of the loops (TADs) generated by head-to-tail and head-to-head pairing in cis between neighboring boundaries is distinct. As illustrated in Figure 1, head-to-tail pairing generates stem-loops, while head-to-head pairing generates circle-loops. The loops could be connected to each other by unanchored loops (Figure 1A and C), or they could be linked directly to each other if boundaries can pair simultaneously with both neighbors (Figure 1B and D). An alternating pattern of TADs connected by DNA segments that crosslink to each other with reduced frequency (c.f., λ DNA below) is not often observed in MicroC experiments. Instead, most TADs appeared to be directly connected to both of their neighbors without an intervening unanchored loop (Batut et al., 2022; Bing et al., 2024; Levo et al., 2022; see also below). This would suggest that TAD boundaries are typically linked to both neighbors, either simultaneously or as alternating pair-wise interactions.
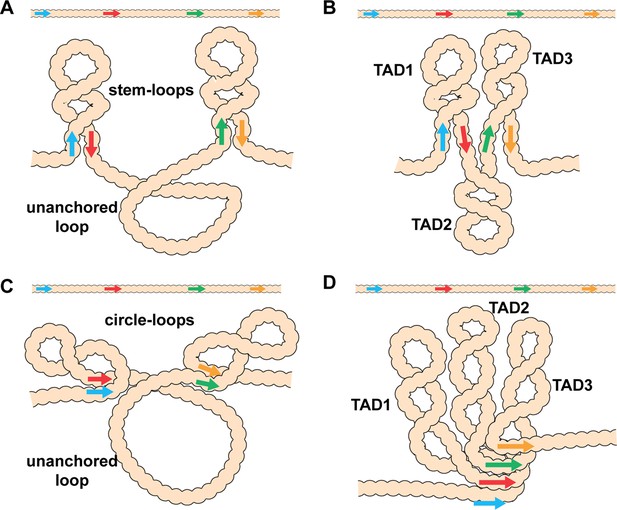
Diagram of the possible loop topologies generated by head-to-head and head-to-tail pairing.
(A) Head-to-tail boundary pairing (arrows) generates a series of stem-loops linked together by an unanchored loop. In this case, the main axis of the chromosome would correspond to the unanchored loops connecting different stem-loops. (B) If boundaries pair with both neighbors (head-to-tail), the stem-loops would be linked to each other by the paired boundaries. In this case the main axis of the chromosome would correspond to the paired boundaries. (C) Head-to-head boundary pairing generates a series of circle-loops linked together by an unanchored loop. The unanchored loop will be the main axis of the chromosome. (D) If boundaries pair with both neighbors (head-to-head), the chromatin fiber will be organized into a series of circle-loops connected to each other at their base, and these paired boundaries will define the chromosomal axis. In both (B) and (D), the pairing interactions between the blue and red boundaries need not be in register with the pairing of the red boundary to the next-door green boundary. In this case, the main axis of the chromosome may bend and twist, and this could impact the relative orientation of the stem-loops/circle-loops. More complex structures would be generated by mixtures of stem-loops and circle-loops.
Key to understanding the 3D organization of chromosomes in multicellular eukaryotes will be the identification of TADs that are stem-loops and TADs that are circle-loops. In the studies reported here, we have used MicroC to analyze the contact maps generated by stem-loops and circle-loops. Stem-loop and circle-loop TADs are expected to interact differently with their neighbors, and this should be reflected in the patterns of crosslinking events between neighboring TADs. As illustrated for linked stem-loops in Figure 1B, TAD2 is isolated from its next-door neighbors, TAD1 and TAD3. In this configuration, crosslinking events between sequences in TAD2 and sequences in TAD1 and TAD3 will be suppressed. On the other hand, TAD1 and TAD3 are in comparatively close proximity, and crosslinking between sequences in these two TADs is expected to be enhanced. A different pattern of neighborly interactions is expected for circle-loop TADs. In this case, the TAD in the middle, TAD2, is expected to interact with both of its neighbors (Figure 1D). To test these predictions, we have first compared the MicroC contact profiles for stem-loop and circle-loop TADs. For stem-loops we selected the eve TAD, while for circle-loops we chose the four TADs that comprise the Abd-B parasegment-specific regulatory domains. We show that these stem-loop and circle-loop TADs have distinctive crosslinking signatures. To confirm these MicroC signatures, we converted the topology of the eve TAD from a stem-loop to a circle-loop. In addition to changing the MicroC signature of the eve TAD, the change in topology is accompanied by changes in the regulatory interactions between eve and its neighbors.
Results
Stem-loops versus circle-loops
The distinctive loop topologies of stem-loops and circle-loops are expected to be reflected in the contact maps that are generated in MicroC experiments. To determine if this is the case, we compared the MicroC contact maps for the eve TAD and the TADs that correspond to the four Abd-B parasegment-specific regulatory domains, iab-5, iab-6, iab-7, and iab-8. The eve TAD is generated by pairing interactions between the nhomie boundary upstream of the eve transcription unit and the homie boundary downstream. Since nhomie and homie pair with each other head-to-tail, the eve TAD has a stem-loop topology (Fujioka et al., 2016). Unlike the eve boundaries, the boundaries that delimit the Abd-B regulatory domains are thought to pair with their neighbors head-to-head (Chetverina et al., 2017; Kyrchanova et al., 2008a; Kyrchanova et al., 2011; Kyrchanova et al., 2008b). This means that the parasegment-specific regulatory domain TADs, iab-5, iab-6, iab-7, and iab-8, are expected to have a circle-loop topology.
As shown in Figure 2, the eve TAD and the four Abd-B TADs have distinctive MicroC contact patterns. The eve TAD is a ‘volcano’ triangle with a plume. The endpoints of the volcano triangle are delimited by nhomie on the left and homie on the right, and within the eve locus (the volcano), there are additional enhanced interactions. While the volcano triangle is generated by contacts between sequences within the eve stem-loop, contacts between sequences in eve and in the neighboring TAD on the left, TL (which contains multiple sub-TADs and six genes: CG15863, CG1418, Pal1, CG12133, eIF3j, and CG12134), are much reduced (L-ev in Figure 2A). There is a similar suppression of contacts between sequences in the eve TAD and sequences in the large neighboring TAD on the right, TM (which contains TER94 and Pka-R2; ev-M in Figure 2A). On the other hand, as expected from the regulatory interactions observed for stem-loops in boundary bypass experiments (Kyrchanova et al., 2008a), physical contacts between sequences in TL and TM are enhanced compared to those between eve and TL (L-ev) or TM (ev-M). Because of the preferential interactions between TADs to either side of the eve stem-loop, the eve volcano triangle is topped by a plume (L-M in Figure 2A). TM also interacts with the two TADs farther to the left of eve, TK (K-M in Figure 2A), and TJ (J-M in Figure 2A; see also Figure 2—figure supplement 1).
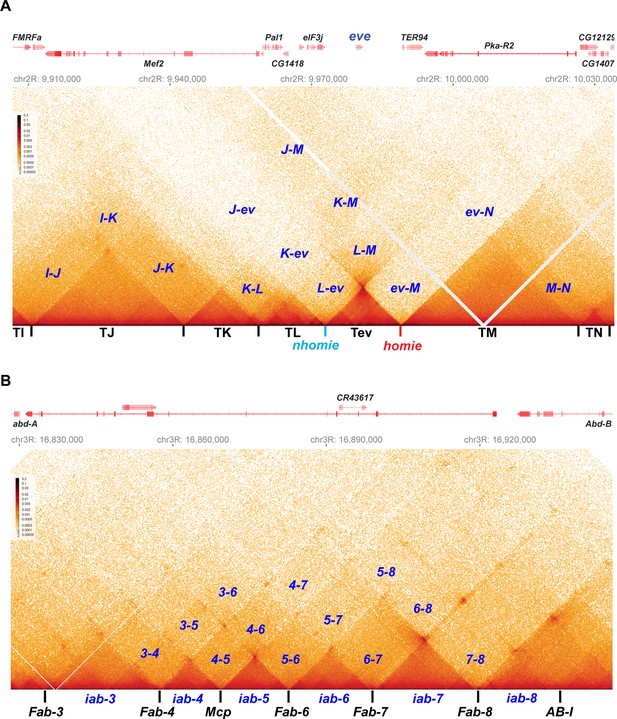
Stem-loops and circle-loops.
“Once in a while you get shown the light in the strangest of places if you look at it right.”a MicroC contact profile for Drosophila wild-type (yw) NC14 embryos. The bin size for each panel is 200 bp. (A) eve and neighboring TADs (TI, TJ, TK TL, TM, and TN). The eve TAD is a volcano with a plume that is anchored by nhomie (nh) and homie (h). The plume is generated by crosslinking of sequences in the two neighboring TADs. At the bottom of the plume, TL sequences are linked to sequences in TM close to eve, including TER94. At the next level, sequences in TK are linked to TM (region K-M). In addition, sequences in TL are linked to sequences in TM located beyond the TER94 gene. At the next level, sequences in TJ are linked to sequences in TM. Note that interactions between sequences in TL and TJ and sequences in TM close to the eve TAD are somewhat less frequent than those farther away from the eve TAD. Sequences in the neighboring TADs also interact with each other, as indicated. For example, sequences in TK and TJ interact with each other (J–K) and also interact with sequences in TI (I–K and I–J). (B) The BX-C gene Abd-B and the parasegment- (PS-) specific regulatory domains iab-3, iab-4, iab-5, iab-6, iab-7, and iab-8. iab-4 regulates the abd-A gene in PS9, while iab-5 – iab-8 regulate Abd-B in PSs 10–13, respectively. These domains are separated from each other by the boundary elements Fab-4, Mcp, Fab-6, Fab-7, and Fab-8, as indicated. The AB-I boundary is located upstream of the Abd-B promoter. Each regulatory domain corresponds to a TAD. Though partially insulated from each other, each TAD interacts with its immediate neighbors. For example, iab-5 interacts with its immediate neighbors iab-4 and iab-6 to give 4–5 and 5–6, respectively. It also interacts with the next-next-door neighbor iab-7 (5-7) and even its next-next-next-door neighbor iab-8 (5-8). (a From ‘Scarlet Begonias’ by the Grateful Dead, 1974).
Like the eve boundaries, the TADs in the Abd-B region of BX-C region are connected to their neighbors by the boundaries at their base. As predicted from genetic studies on BX-C boundaries, each TAD corresponds to one of the four parasegment-specific regulatory domains. While the MicroC contact maps for the four Abd-B TADs resemble the contact patterns in the eve TAD, these Abd-B TADs differ from eve in that there are no plumes above their triangle peaks (Figure 2B). Instead, the Abd-B TADs are overlaid by a series of rectangular interlocking low-density contact (LDC) domains, or clouds. As illustrated in Figure 2B, the iab-6 regulatory domain is flanked by clouds generated by crosslinking with its next-door neighbors iab-5 (5–6) and iab-7 (6–7), followed by crosslinking with neighbors that are a TAD away from iab-6, iab-4 (4–6), and iab-8 (6–8). The other regulatory domains also form a unique set of interlocking LDCs/clouds with their immediate neighbors, their next-next-door neighbors, and their next-next-next-door neighbors.
TAD formation in a nhomie deletion
To further investigate the pairing properties and functioning of the eve boundaries, we used CRISPR-Cas9 to add two attP sites flanking the nhomie region, replacing the region with a mini-white gene. Using mini-white as an exchange marker, ΦC31 recombinase-mediated cassette exchange (RMCE) was used to restore the sequence of the region, with nhomie modifications. As a control for possible effects of the sequences introduced in generating the modification, we reinserted a 597 bp nhomie DNA fragment in the same orientation as the endogenous nhomie boundary (nhomie forward). To maintain roughly the same distance between eve and the neighboring TAD in the nhomie deletion, we introduced a 606 bp DNA fragment from phage λ (λ DNA). Figure 3 (and Figure 2—figure supplement 1A and B) shows the MicroC contact profiles for the nhomie forward and λ DNA replacements in 12–16 hr embryos (mid-embryogenesis: stages 12–14). Except that the sequencing depth of the nhomie forward replacement is not as great as the WT shown for the eve locus in Figure 2, the profile is quite similar. Like WT, there are sub-TADs within the eve TAD. One of these appears to link homie and the neighboring PRE to the eve promoter-proximal PRE (Fujioka et al., 2008), and is marked by an interaction dot (asterisk in Figure 3A). Another links nhomie to the eve promoter region (blue arrow in Figure 3A). The eve TAD is topped by a plume, which is generated by interactions between sequences in neighboring TADs TL with TM (L-M). On the other hand, interactions between eve and its neighbors are suppressed. Like eve, there are sub-TADs in the neighboring TAD, TL. The TL sub-TAD closest to eve (TL4) corresponds to the CG12134 transcription unit (green arrowhead marks the boundary: Figure 3), while the neighboring sub-TAD (TL3) encompasses the eIF3j transcription unit (blue arrowhead: Figure 3).
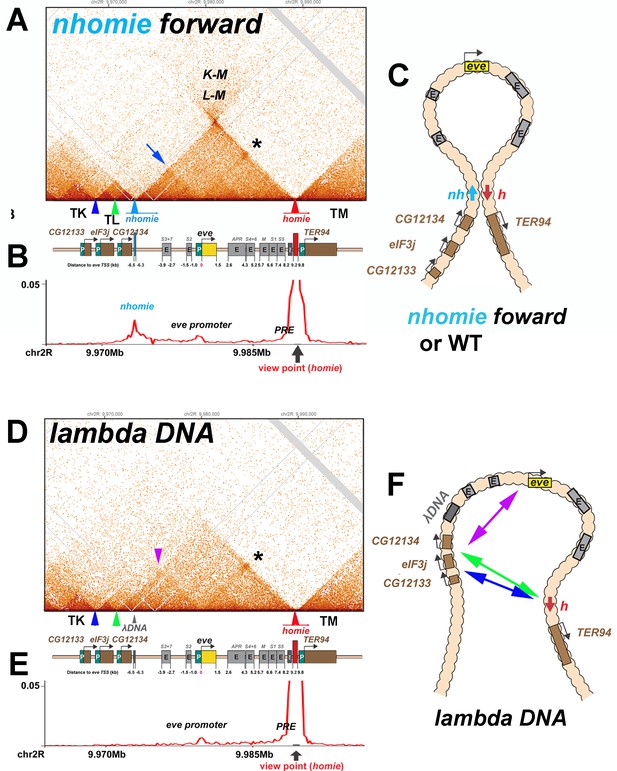
TAD organization of the nhomie forward and lambda DNA replacements.
(A) MicroC contact profile of 12–16 hr embryos (stage 12–14) nhomie forward embryos. In this, our positive control, nhomie replaces endogenous nhomie, in the same orientation. N (replicates) = 2. Resolution = 200 bp. L-M: interactions between TADs TL and TM flanking the eve locus. Asterisk: sub-TAD linking the eve promoter to the eve PRE and homie. Dark blue arrow: sub-TAD linking the eve promoter to nhomie. Light blue arrow: nhomie. Red arrow: homie. Green arrowhead: sub-TAD boundary formed by the CG12134 promoter region. Dark blue arrowhead: sub-TAD boundary formed by eIF3j promoter region. Diagram: map of eve locus and surrounding genes. (B) Virtual 4C with viewpoint from homie (black arrow) in nhomie forward embryos. (C) Diagram of the eve stem-loop TAD. (D) MicroC contact profile of 12–16 hr λ DNA embryos. In this replacement, λ DNA is inserted in place of nhomie. N (replicates) = 3. Resolution = 200 bp. Asterisk: sub-TAD linking the eve promoter to the eve PRE and homie. Purple arrowhead: sub-TAD linking CG12134 promoter region to the eve promoter. The eIF3j sub-TAD TL3 (between the blue and green arrowheads) is still present. (E) Virtual 4C with viewpoint from homie (black arrow) in λ DNA embryos. (F) Diagram of the ‘unanchored’ eve TAD. Double arrows show novel interactions.
The MicroC profile of the λ DNA replacement (Figure 3D) is quite different from that of either nhomie forward or WT, which are similar (Figures 2A and 3A). While homie still defines the distal (relative to the centromere) end of the eve locus, the λ DNA replacement does not function as a TAD boundary, and the leftward endpoint of the eve TAD is no longer well-defined. One new ‘endpoint’ for the eve locus maps to sequences between CG12134 and eIF3j (green arrowhead), which in wild type corresponds to the left boundary of the TL sub-TAD TL4. The other endpoint maps to sequences between eIF3j and CG12133 (blue arrowhead), which in wild type define the left boundary of the TL sub-TAD TL3. These interactions are not as stable as those between nhomie and homie as the density of interaction dots is lower. Furthermore, they appear to flip back and forth between alternative endpoints (as indicated by the green and blue double arrows in Figure 3F) based on the MicroC contact profile, which is consistent with a mixture of (at least) two conformations. The eve TAD also interacts with sequences in the two other TL sub-TADs, TL1 and TL2. In addition, the eve promoter appears to interact with sequences located upstream of CG12134 (purple arrowhead in Figure 3D and double arrow in Figure 3F), while this interaction is not observed in the nhomie forward replacement. While the TL TAD (from the TK:TL boundary to nhomie) is also disrupted by the nhomie deletion (it has a much less distinct ‘volcano apex’, and its right-most sub-TAD TL4 is now fused with the eve TAD), the left-most TL sub-TADs (TL1, TL2, and TL3) are still present, indicating that their formation does not depend on nhomie. As shown in the virtual 4C at homie viewpoint in Figure 3B and E, the homie boundary interacts with the nhomie forward replacement, but does not contact the λ DNA replacement.
eve enhancers activate eIF3j expression in the nhomie deletion
In transgene assays, boundary elements block regulatory interactions when interposed been enhancers (or silencers) and reporter genes (Chetverina et al., 2014; Chetverina et al., 2017; Kellum and Schedl, 1992). To determine whether this is also true in their endogenous context, we compared the expression in syncytial blastoderm embryos of the two genes that flank nhomie at the eve locus, eIF3j and CG12134. As the nhomie deletion eliminates homie’s pairing partner and disrupts the eve TAD, we also examined the expression of eve and of the gene just beyond the homie boundary, TER94, which has strong maternal expression through stage 11 (Figure 4—figure supplement 2B, WT). Consistent with the seemingly normal MicroC profile on the homie side of the eve TAD, we did not detect evidence of eve-like TER94 expression (Figures 4 and 5). Thus, the formation and functioning of the TM TAD do not appear to be impacted by either the loss of nhomie per se or the fact that the left end of the eve TAD is no longer properly anchored.
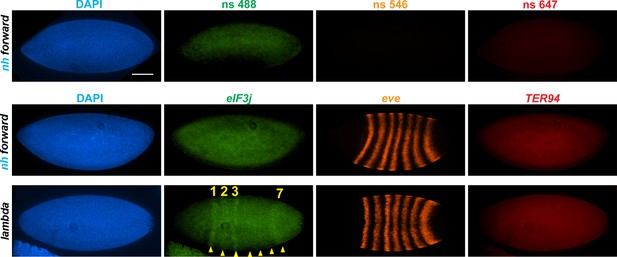
nhomie deletion (λ DNA replacement) exposes eIF3j to the eve enhancers.
nh forward: positive control, as in Figure 3. λ DNA: nhomie is replaced with λ DNA. At the syncytial blastoderm stage, a series of stripe-specific enhancers upstream (stripes 1, 2, 3, 7) and downstream (stripes 1, 4, 5, 6) of the eve gene drive eve expression. During cellularization of the blastoderm and gastrulation, a single enhancer located upstream of eve drives expression of all seven stripes. DAPI: DNA stained with DAPI (blue). eIF3j: embryo hybridized with probe complementary to eIF3j mRNA. eve: embryo hybridized with probe complementary to eve mRNA. TER94: embryo hybridized with probe complementary to TER94. Yellow arrowheads: eve-enhancer-driven eIF3j stripes. Control nonspecific probes for each channel indicate autofluorescence background in the top panel. Scale bar = 100 µm.
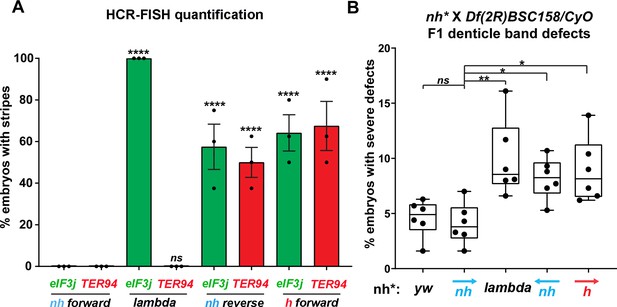
Manipulating the nhomie boundary impacts the regulatory landscape.
N = # of independent replicates, n = # of embryos. Two-way ANOVA with Tukey’s multiple comparisons test for each pair of groups was used to determine the statistical significance. *p≤0.05, **p≤0.01, ***p≤0.001, and ****p≤0.0001. (A) Quantitation of the number of embryos showing stripe patterns in HCR-FISH for eIF3j and TER94, as shown in Figures 4 and 7. N = 3. n = 45 for each group. (B) Quantitation of the number of missing ventral denticle bands in larvae from a cross of BSC/CyO,hb-lacZ deficiency females to males of the indicated genotypes (N = 6): wild-type control (yw), n = 767. For the nhomie forward replacement, n = 1099; for the λ DNA replacement, n = 1175; for the nhomie reverse replacement, n = 1083; for the homie forward replacement, n = 1137.
In the case of the gene closest to the nhomie deletion, CG12134, we were unable to consistently detect transcription driven by the eve enhancers in either nhomie forward or λ DNA embryos. In some λ DNA embryos, there were hints of stripes at the blastoderm stage (see Figure 4—figure supplement 1); however, these ‘stripes’ were not observed in most embryos. Since CG12134 (which forms the TL4 sub-TAD in wild type) is closest to the eve enhancers and interacts most strongly, it is possible that the promoter is not compatible with the eve enhancers. A different result was obtained for eIF3j in λ DNA embryos. As shown in the HCR-FISH experiment in Figure 4 and quantitated in Figure 5, we observed a series of eIF3j stripes over a dark background in pre-cellular blastoderm embryos. As this background hybridization is evident in earlier stages, much of it is likely to be of maternal origin. In contrast to the λ DNA replacement, these stripes are not visible in the nhomie forward (control) replacement (Figures 4 and 5A). While it is possible to detect all seven stripes in λ DNA blastoderm stage embryos, their levels of expression are not equal. The highest levels correspond to eve stripes 1, 2, 3, and 7, while eve stripes 4, 5, and 6 are expressed at much lower levels. Since the stripe enhancers for 1, 2, 3, and 7 are located between the eve promoter and nhomie, they are closer to the eIF3j promoter than the enhancers for stripes 4, 5, and 6, which are located downstream of the eve transcription unit. In addition to possible effects of distance, the subdomain linking the eve PRE and homie to the promoter is still observed in the λ DNA replacement, and this could partially sequester the enhancers located downstream of the eve promoter.
We also used digoxigenin in situ hybridization to analyze eIF3j expression. With this procedure we were able to detect a low level of eve-activated eIF3j stripe expression in WT and nhomie forward embryos at stage 5 (blastoderm) and stages 7–8 (early gastrula: Figure 4—figure supplement 2A). In stage 5 embryos when eve expression is driven by specific stripe enhancers, eIF3j expression appears to be similar in all seven stripes (Figure 4—figure supplement 2, WT and nhomie forward). In contrast, as was observed in the HCR-FISH experiments (Figure 4), there is a clear bias for enhancers located upstream of eve, where eIF3j is also located, in the λ DNA replacement at this point in development. In stage 7–8 embryos, the seven-stripe enhancer drives eve expression. It is located close to nhomie and, not surprisingly, high levels of eIF3j expression are observed in all seven stripes in the λ DNA replacement (Figure 4—figure supplement 2A). At later embryonic stages, eve expression is driven by tissue-specific neurogenic, mesodermal, and anal plate enhancers. However, eIF3j is expressed at high levels in a complex pattern in older embryos, and we were unable to unambiguously detect expression driven by the eve enhancers over this background mRNA. This is also not surprising, given that all of the enhancers for these aspects of eve expression are located downstream of the eve promoter, like the enhancers driving stage 5 stripes 4, 5, and 6.
While the nhomie deletion did not have any obvious impact on the level or pattern of eve expression in blastoderm stage embryos (see Figure 4), it seemed possible that eve activity was not entirely normal. To test this possibility, we mated males homozygous for either λ DNA or nhomie forward to females heterozygous for a chromosomal deficiency that includes the eve gene. We then quantitated the number of missing denticle bands in embryonic cuticle preps. As shown in Figure 5B, the frequency of larvae with ‘severe’ defects (two or more missing ventral denticle bands) in nhomie forward is similar to that in a WT yw control. In contrast, in the λ DNA replacement, the frequency of larval cuticles with two or more missing denticle bands is increased nearly twofold. Taken together, the increase in severity of the cuticle defects is significant at the p<0.01 level (one-tailed t-test). The A6 denticle band is missing most frequently, followed by A2, A4, and then A8. These even-numbered abdominal denticle bands are those that are lost in eve deficiency mutants (from which the name even skipped comes), suggesting that eve stripe expression at blastoderm stages is compromised in the embryos that produce these defective cuticles.
The eve TAD is converted from a stem-loop to a circle-loop by inverting nhomie
The orientation of boundary:boundary pairing interactions determines the topology of each chromatin loop (Bing et al., 2024; Fujioka et al., 2016). Since nhomie and homie pair with each other head-to-tail, the endogenous eve TAD is a stem-loop. This orientation dependence means that one can convert the eve TAD from a stem-loop to a circle-loop by inverting the nhomie boundary. If our expectations are correct, the MicroC contact pattern will also be transformed from a volcano triangle with a plume to one in which sequences in the eve TAD are flanked by a cloud of crosslinked sequences from both neighboring TADs (TL and TM), like that observed in the Abd-B region of the BX-C.
We tested this prediction by inserting the nhomie boundary in the reverse orientation (nhomie reverse). Figure 6A shows that the eve TAD is reconstituted by nhomie reverse (compare with Figure 3: see also Figure 2—figure supplement 1). The sub-TAD evident in the nhomie forward replacement linking nhomie to the eve promoter is also re-established (blue arrow). In addition, consistent with our expectation, the plume topping the eve TAD is gone and is replaced by a much more sparsely populated LDC domain (purple double-arrow and above). The more prominent LDC TAD-TAD interactions (the clouds) are between sequences in the eve TAD and the neighboring TADs. On the right, eve forms an LDC interaction domain with TM (ev-M). On the left, eve interacts most strongly with sequences in TL4, and progressively less strongly with sequences in the sub-TADs TL3, TL2, and then TL1 (L-ev). In addition to restoring the eve TAD, the TL TAD is re-established, indicating that the nhomie boundary is important in defining both endpoints of the TL TAD. On the other hand, with the exception of the CG12133 sub-TAD, nhomie does not play a role in generating the three other sub-TADs in the TL TAD. The other interesting feature is a 45o band of interaction (just below the purple double-arrow) that includes interactions between sequences in TL4 and sequences in eve that appear to be located near the left edge of homie. These sequences likely correspond to the eve 3′ PRE (Fujioka et al., 2008), located just inside the 3′ end of the eve TAD.
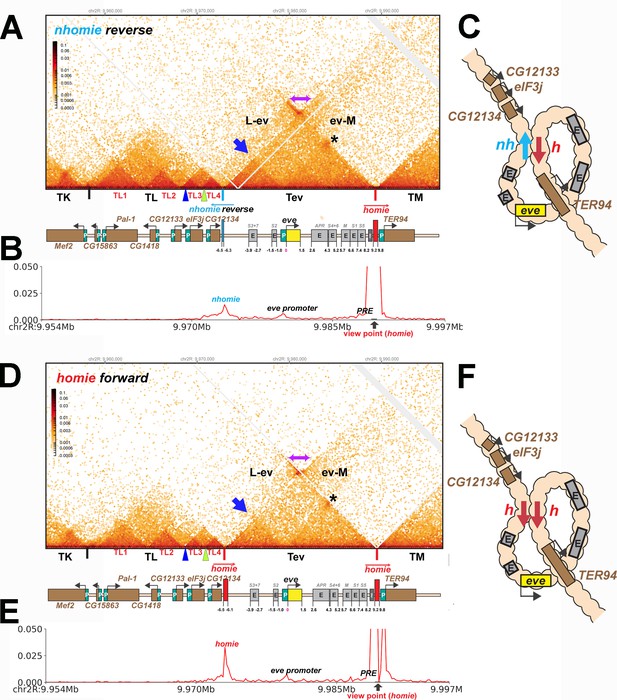
TAD organization of the nhomie reverse and homie forward replacements.
(A) MicroC contact profile of 12–16 hr nhomie reverse embryos. In this replacement, nhomie is inserted in the reverse orientation compared to WT nhomie. N (replicates) = 3. Resolution = 200 bp. (B) Virtual 4C with viewpoint from homie (black arrow) in nhomie reverse embryos. (C) Diagram of the nhomie reverse:homie circle-loop. (D) MicroC contact profile of 12–16 hr homie forward embryos. In this replacement, homie is inserted in the forward orientation (the same as the endogenous homie): N (replicates) = 3, resolution = 200 bp. (E) Virtual 4C with viewpoint from homie (black arrow) in homie forward embryos. (F) Diagram of the homie forward:homie circle-loop. (A, C) Note that interactions between the TADs flanking the eve locus (purple double arrow) are suppressed compared to nhomie forward (see Figure 3), while interactions of the eve TAD (Tev) with TL and TM are enhanced (L-ev and ev-M). Asterisk: sub-TAD linking the eve promoter to the eve PRE and homie. Dark blue arrow: sub-TAD linking the eve promoter to nhomie reverse. Light blue arrow: nhomie reverse. Red arrow: homie. Green arrowhead: sub-TAD boundary formed by the CG12134 promoter region. Dark blue arrowhead: sub-TAD boundary formed by the eIF3j promoter region.
Insulation is reduced in nhomie reverse
Consistent with the models for stem-loops and circle-loops in Figure 1, the neighborly interactions evident in the MicroC contact patterns for nhomie forward and nhomie reverse are quite distinct. The nhomie forward TAD is isolated from its neighbors, and crosslinking between eve and the neighboring TADs is suppressed (Figures 1B and 2A). This is not true for the circle-loop TAD generated by nhomie reverse: in this configuration, the eve TAD is not sequestered from neighboring TADs (Figures 1D and 2B), but instead interacts much more frequently with sequences in next-door TADs than in the stem-loop configuration. Since the eve TAD is no longer as well-isolated from its neighbors, this could increase the frequency of ‘productive’ interactions between eve regulatory elements and genes in nearby TADs, and vice versa.
To test whether the circle-loop topology has an impact on the regulatory landscape, we hybridized nhomie reverse embryos with HCR-FISH probes for eIF3j, TER94, and eve. In early blastoderm stage nhomie forward embryos, there is little evidence of eIF3j or TER94 expression driven by eve stripe enhancers, and the HCR-FISH hybridization pattern is uniform (see Figure 4). In contrast, it is possible to discern individual stripes of both eIF3j and TER94 mRNA over the background signal in a subset of nhomie reverse blastoderm stage embryos in HCR-FISH (Figure 7). Since these genes are assembled into their own topologically independent looped domains rather than being in the same domain as eve, the level of eIF3j and also TER94 stripe expression is lower than that observed in the nhomie deletion (λ DNA). The nhomie reverse circle-loop also differs from the nhomie deletion (λ DNA) in that there is not such an obvious preference for which eve enhancers activate expression. In addition, eve-dependent eIF3j and TER94 stripes are detected in only about half of the blastoderm stage embryos (Figure 5A). It is possible that the frequency of productive inter-TAD contacts differs from one embryo to the next; however, a more likely reason is that the high background of eIF3j and TER94 transcripts obscures the low level of eve enhancer-driven expression at this stage. Once the seven-stripe enhancer is activated, eve-dependent TER94 expression in nhomie reverse is elevated, and all seven stripes are observed (Figure 4—figure supplement 2B). This fits with the MicroC contact profile. As shown in Figure 6A, the TER94 gene is preferentially crosslinked to eve sequences located between nhomie reverse and the eve promoter compared to sequences spanning the eve gene and the downstream enhancers (i.e., the upper-left portion of the ev-M region shows more crosslinking than does the lower-right portion). This bias correlates with the location of the seven-stripe enhancer, located near the 5′ end of the eve TAD. By contrast, there is much less seven-stripe enhancer-driven expression of eIF3j (Figure 4—figure supplement 2A) than of TER94. Consistent with this observation, crosslinking between eIF3j and sequences in eve close to nhomie reverse and the seven-stripe enhancer occur less frequently than crosslinking to sequences on the other side of the eve TAD (i.e., the lower-left portion of the L-ev region of the MicroC profile shows less crosslinking than does the upper-right portion), although this crosslinking bias may not be as pronounced as that observed between TER94 and the two sides of the eve TAD.
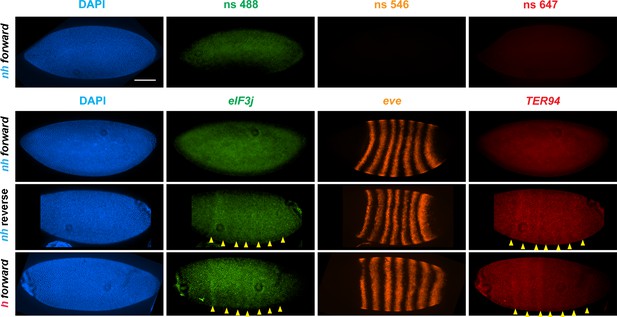
eve enhancers activate neighboring genes when the eve TAD is a circle-loop.
HCR-FISH hybridization to mRNA expressed by eIF3j, eve and TER94 at the blastoderm stage (embryonic stage 5). nh forward: nhomie is replaced with nhomie in the forward (normal) orientation (positive control, as in Figure 3). nh reverse: nhomie is replaced with nhomie in the reverse orientation. h forward: nhomie is replaced with homie in the forward orientation. Yellow arrowheads: positions of stripes. DAPI (blue): DNA stained with DAPI. eIF3j (green): embryo is hybridized with probe complementary to eIF3j mRNA. eve (orange): embryo is hybridized with probe complementary to eve mRNA. TER94 (red): embryo is hybridized with probe complementary to TER94. Control nonspecific probes for each channel indicate autofluorescence background in the top panel. Scale bar = 100 µm.
While eve stripe expression is not discernibly different from wild type, the circle-loop topology still impacts eve function. As shown in Figure 5B, the fraction of nhomie reverse embryos with two or more missing denticle bands is nearly twice that in either yw or the nhomie forward replacement. Taken together, the increase in severity of the cuticle defects is significant at the p<0.05 level (one-tailed t-test). As was observed for the λ DNA replacement, the A6 denticle band is missing most frequently, followed by A2, A4, and then A8.
homie forward converts the eve stem-loop into a circle-loop
While the findings in the previous section show that loop topology impacts how sequences in TADs interact with each other and with their neighbors, one might argue that the effects we observed are a reflection of some novel properties of the nhomie boundary when it is inverted. To test this possibility, we took advantage of the fact that in addition to pairing with nhomie, the homie boundary pairs with itself (Fujioka et al., 2016). However, unlike nhomie:homie pairing, which is head-to-tail, homie:homie pairing is head-to-head. This means that it is possible to convert the eve TAD into a circle-loop by inserting homie into the nhomie deletion in the forward orientation.
As shown in Figure 6D (Figure 2—figure supplement 1), the MicroC contact profile of homie forward is similar to that observed for nhomie reverse. The plume topping the eve TAD in wild type (Figure 2A) or in nhomie forward (Figure 3A) is absent. Likewise, instead of being isolated from its neighbors, the eve TAD contacts TL and TM. Also like nhomie reverse, homie forward forms a subdomain within the eve TAD linking it to sequences in the eve promoter. There is also enhanced crosslinking between sequences in TL4 and sequences on the right end of the eve TAD that correspond to the eve 3′ PRE (Fujioka et al., 2016).
The MicroC pattern is not the only similarity between homie forward and nhomie reverse. The functional properties of the homie forward eve TAD are also similar. Figure 7 shows that the eve enhancers weakly activate both eIF3j and TER94. As was the case for nhomie reverse, expression levels at the blastoderm stage are low and are observed in only about half of the embryos (Figure 5A). After the blastoderm stage, when the seven-stripe enhancer drives eve expression, an even higher level of TER94 expression is observed (Figure 4—figure supplement 2B). In addition, the functioning of the eve gene when it is in the circle-loop configuration is not as efficient, and the frequency of homie forward embryos with two or more missing denticle bands is twice that of nhomie forward (Figure 5B). Taken together, the increase in severity of the cuticle defects is significant at the p<0.05 level (one-tailed t-test).
Discussion
Two different though overlapping classes of chromosomal architectural elements have been identified in flies. One class is the PREs found in many developmental loci. PREs were first discovered because they induce pairing-sensitive silencing of reporter genes (Americo et al., 2002; Kassis et al., 1991). More recent studies have shown that the ability of these elements to physically pair with each other may be their most important function (Batut et al., 2022; Levo et al., 2022). The other class of architectural elements are chromatin boundaries (also called insulators). PRE pairing in cis typically takes place within the context of a larger chromosomal domain, or TAD. In contrast, boundary elements are responsible for defining the endpoints of these looped domains (Arzate-Mejía et al., 2020; Batut et al., 2022; Bing et al., 2024; Chetverina et al., 2017; Ibragimov et al., 2023; Stadler et al., 2017). While not much is known about the parameters governing PRE pairing, the pairing interactions of fly boundaries have been studied in some detail. The key features include an ability to engage in promiscuous pairing interactions, distinct partner preferences, and orientation dependence. Of the endogenous (non-gypsy) boundaries whose functional properties have been studied in detail, only one, Fab-7, appears to be able to pair in both orientations. However, Fab-7 may be unusual in that its boundary activity depends upon factors that have been implicated in the functioning of PREs (Kyrchanova et al., 2018). For all of the other boundaries studied so far, pairing interactions are orientation-dependent. When fly boundaries pair with themselves, the interactions are head-to-head (Kyrchanova et al., 2008a). This make sense, as the available evidence suggests that self-pairing interactions in trans may be largely responsible for the pairing of homologous chromosomes in precise register (Erokhin et al., 2021; Fujioka et al., 2016). In this case, head-to-tail self-pairing would uncouple the loops on the two homologs (and sister chromatids).
Unlike self-pairing in trans, pairing interactions between heterologous boundaries in cis can be head-to-head or head-to-tail. The topological consequences are quite distinct. The former generates a circle-loop, while the latter forms a stem-loop (Chetverina et al., 2017). In the studies reported here, we have investigated how these two different topologies impact the local chromatin organization. We have also determined whether circle-loops and stem-loops alter the ability of boundary elements to define units of independent gene activity and insulate against regulatory interactions between neighboring TADs.
nhomie deletion disrupts the eve TAD
As would be predicted from many different studies (Cavalheiro et al., 2021; Chetverina et al., 2017), deletion of the nhomie boundary and replacement with a control λ DNA disrupts the eve TAD and alters the regulatory landscape. The MicroC profile shows that disruption of the eve TAD and the neighboring TADs is one-sided. Within the eve TAD, the subdomain linking homie and the nearby PRE to the eve promoter is unaffected. Likewise, the large TAD, TM, which encompassess both TER94 and pka-R2, and is defined at one end by homie and at the other by an uncharacterized boundary element upstream of the pka-R2 promoter, is intact (Figure 2—figure supplement 1B). In contrast, on the nhomie side of the eve TAD, the sub-TAD linking nhomie to the eve promoter is absent and is replaced by a less well-defined sub-TAD linking the eve promoter to an element near the CG12134 promoter. However, the endpoint of the eve TAD is no longer distinct, and eve sequences are now crosslinked to the eIF3j sub-TAD and to sequences in the more distant TL sub-TADs TL3, TL2, and TL1. Consistent with these alterations in the physical organization of the eve and neighboring TADs, the TER94 gene is still insulated from the eve enhancers. While sequences in the eve TAD physically interact with the gene closest to nhomie, CG12134, only the next gene over, eIF3j, is clearly activated by the eve enhancers. Since crosslinking between sequences in CG12134 and the eve TAD is more frequent than crosslinking between the eIF3j sub-TAD TL3 and the eve TAD, it seems likely that CG12134 is refractory to activation by the eve enhancers. This could be due to an incompatibility between the CG12134 promoter and the eve enhancers. Alternatively, the promoter may not be active at this stage. While eIF3j is activated by the eve enhancers in stage 5 embryos in the nhomie deletion, the stripe enhancers located upstream of the eve gene drive a higher level of expression than those located downstream. Two factors in addition to the effects of distance could potentially account for this finding. Since the eve promoter is located between the downstream enhancers and the eIF3j gene, activation of eIF3j by the downstream enhancers could be suppressed by promoter competition. Alternatively, or in addition, the sub-TAD formed between the 3′ PRE/homie and the eve promoter/proximal PRE (Fujioka et al., 2008) could tend to isolate the downstream eve stripe enhancers from interactions with eIF3j.
Topology impacts local 3D genome organization and the potential for regulatory interactions
In boundary bypass experiments using endogenous fly boundaries, the ability of the upstream enhancers to activate the downstream reporter depended on the topology of the loop generated by the paired boundaries (Kyrchanova et al., 2008a). Activation is observed for stem-loops, as this configuration brings the upstream enhancers into close proximity with the downstream reporter. In contrast, the enhancers and downstream reporter are not brought into contact when the topology is a circle-loop. As would be predicted from these bypass experiments, the stem-loop formed by the head-to-tail pairing of nhomie and homie physically isolates the eve TAD from its neighbors, and this is reflected in the low density of contacts between sequences in eve and the neighboring TADs (Figure 2A). Conversely, the TADs that flank eve are brought together, and contacts between them generate the plume that is observed above the eve volcano triangle.
The physical isolation afforded by the stem-loop topology is lost when the eve TAD is converted to a circle-loop either by inverting the nhomie boundary or by replacing nhomie with the homie boundary inserted in the forward direction (Figure 6). In the former case, head-to-tail nhomie:homie pairing generates a circle-loop. In the latter case, head-to-head pairing of homie (inserted in the forward orientation) with endogenous homie generates a circle-loop. The alteration in the local 3D organization induced by the conversion of eve to a circle-loop is evident from the changes in the MicroC contact pattern. Instead of being isolated from neighboring TADs, the eve TAD interacts not only with its immediate neighbors, but also with more distant TADs. As a result, the plume of enhanced contacts linking TM to TL, TK, and TJ is absent and is replaced by contacts between these TADs and the eve TAD.
As might be expected from the MicroC contact patterns, the conversion to a circle-loop topology is accompanied by alterations in regulatory interactions between eve and the genes in the neighboring TADs (Figure 7, Figure 4—figure supplement 2). Unlike the nhomie forward replacement, the eve stripe enhancers in both of the circle-loop replacements are able to weakly activate expression of two neighboring genes, eIF3j and TER94. This pattern of activation mirrors the enhancement in contacts between the eve TAD and the neighboring TL and TM TADs evident in MicroC experiments. Thus, though eIF3j and TER94 are clearly shielded from the eve enhancers when the eve TAD is a circle-loop, a greater degree of isolation from the action of the eve enhancers is afforded when the eve TAD is a stem-loop.
In addition to reducing insulation from regulatory interactions with genes in neighboring TADs, the circle-loop topology impacts the functioning of the eve gene (Figure 5B). For both nhomie reverse and homie forward, the frequency of multiple denticle band defects compared to the nhomie forward control is enhanced in a sensitized genetic background. While we did not detect any obvious reductions in the eve stripes in blastoderm stage embryos, the circle-loop provides less insulation than the stem-loop, and it is possible that the neighboring promoters suppress eve expression by competing for the eve enhancers. Another (nonmutually exclusive) possibility comes from the studies of Yokoshi et al., 2020, who used live imaging to examine the effects of flanking a reporter with the nhomie and homie boundaries. In their experiments, reporter expression was enhanced over twofold when the reporter was flanked by nhomie and homie; however, the enhancement was greater when the paired boundaries formed a stem-loop than when they formed a circle-loop.
Boundary:boundary pairing can generate stem-loops and circle-loops
Our manipulations of the nhomie boundary support the notion that TADs can have two different loop topologies, stem-loop and circle-loop, and these topologies impact their physical and biochemical properties. Since circle-loop TADs cannot be generated in the popular cohesin loop extrusion/CTCF roadblock model for the sculpting the 3D genome, it would be important to know whether there are other unambiguous examples of loops with either a stem-loop or circle-loop topology besides those described here. As discussed above, the available evidence suggests that the boundaries in the Abd-B regulatory domains pair with their neighbors head-to-head, and thus form circle-loops. While the contact pattern between neighbors in the Abd-B region fit with that expected for an array of circle-loop TADs, this has not been confirmed by examining the MicroC profiles before and after manipulating the boundary elements in this region. For this reason, we sought unambiguous examples of chromatin loops generated by the orientation-dependent pairing of endogenous TAD boundaries that have either a stem-loop or a circle-loop topology. The collection of meta-loops described recently by Mohana et al., 2023 provide one such test, as many appear to be generated by the pairing of TAD boundaries, and their local interaction profiles are easily interpreted.
Shown in Figure 8A is a 2.8 Mb meta-loop on chromosome 2L generated by the pairing of two TAD boundaries, labeled blue and purple (block arrows). The pairing of the blue and purple TAD boundaries brings sequences in the TADs flanking the two boundaries into contact, and this generates two rectangular boxes of interaction indicated by the arrows (see also blue double arrows in the diagram on the right). In the rectangular box on the upper left of the contact map, sequences in the TAD containing CG33543, Obp22a, and Npc2a located just upstream of the blue boundary are crosslinked to sequences upstream of the purple boundary in the TAD containing the fipi gene. In the rectangular box on the lower right, sequences in a small TAD downstream of the blue boundary (which contains Nplp4 and CG15353) are crosslinked to sequences in a TAD downstream of the purple boundary (which contains CG3294 and slf). As shown in the diagram, this pattern of interaction (upstream-to-upstream and downstream-to-downstream) indicates that the blue and purple TAD boundaries pair with each other head-to-head, and this orientation generates a large loop with a circle-loop topology.
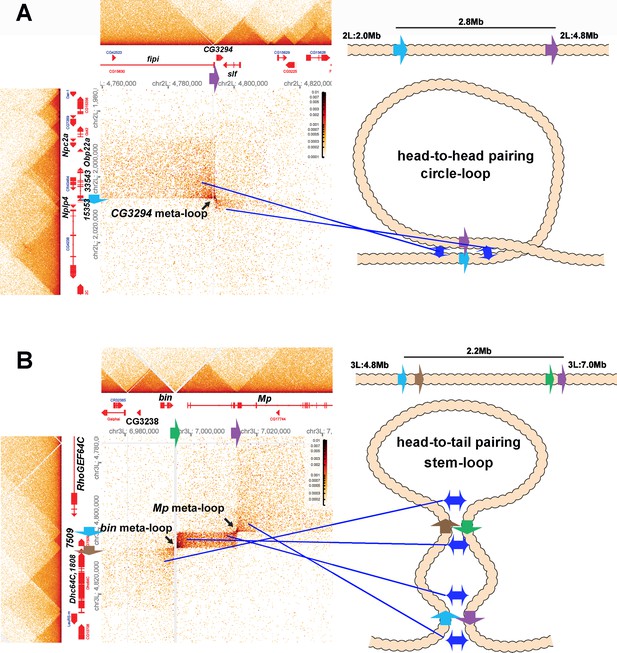
Circle-loop and stem-loop meta-loops.
(A) CG3294 circle-loop meta-loop. In this meta-loop, a TAD boundary (blue arrow) located at ~2.0 Mb on chromosome 2L pairs head-to-head with a TAD boundary (purple arrow) located ~2.8 Mb away. As indicated in the diagram, head-to-head pairing generates a circle-loop. In the circle-loop topology, the TAD upstream of the blue boundary is brought into contact with the TAD upstream of the purple boundary, as indicated the diagram (blue double arrows). This generates a rectangular box of enhanced contacts between sequences in the TAD containing the CG33543, Obp22a, and Npc2a genes and sequences in a TAD that contains the fipi gene. This box is located on the upper left of the contact map (above and to the left of the black arrow). Sequences in TADs downstream of the blue and purple boundaries are also linked, and this generates a small rectangular box representing sequences in the small Nplp4 and CG15353 TAD ligated to sequences in the TAD containing CG3294 and slf (below and to the right of the black arrow). (B) The bin/Mp meta-loops on the left arm of the third chromosome are generated by the head-to-tail pairing of two sets of boundaries, indicated by the blue, brown, green, and purple arrows. Pairing of the brown and green boundaries generates an ~2.2 Mb stem-loop. Sequences in the TAD downstream of the brown boundary (which contains the Dhc64C and CG1808 genes) are linked to sequences in the TAD upstream of the green boundary (which contains CG2328 and bin). This generates the rectangular box of enhanced contacts on the lower left of the contact map. Pairing of the blue and purple boundaries head-to-tail generates a small stem-loop ‘bubble’ (see diagram). This bubble brings sequences in the TAD containing the most distal Mp promoter (blue arrowhead) into contact with sequences in the small TAD containing CG7509 (see diagram on the right). Interactions between these two TADs generates the small rectangular box of enhanced contacts in the center of the contact map. The head-to-tail pairing of the blue and purple boundaries also bring sequences in the TAD upstream of the blue boundary that contains the RhoGEF64C gene into contact with the TAD containing one of the internal Mp promoters (black arrowhead). This interaction generates the box of enhanced contacts in the upper-right portion of the contact map. The bin size for each panel is 200 bp; embryos are 12–16 hr old.
The 2.2 Mb meta-loop on chromosome 3L in Figure 8B is more complicated in that it is generated by four TAD boundaries (indicated by blue, brown, green, and purple arrows). The blue and brown boundaries separate a small TAD containing CG7509 from two larger TADs, while the green and blue boundaries define the endpoints of a TAD containing the most distal promoter (blue arrowhead) of the Mp (Multiplexin) gene. As indicated in the accompanying diagram, the brown and green boundaries pair with each other head-to-tail as do the blue and purple boundaries. Pairing of the brown and green boundaries generates a large ~2.2 Mb stem-loop that brings sequences in the TAD downstream of the brown boundary, which contains the Dhc64C and CG1808 genes, into contact with sequences in the TAD upstream of the green boundary, which contains the CG3238 and bin (binou) genes. This linkage generates the rectangular box of enhanced contacts in the lower-left corner of the contact map. Pairing of the blue and purple boundaries generates a small stem-loop bubble that links sequences in the CG7509 TAD to the TAD containing the Mp distal promoter (blue arrowhead). This connection generates a small rectangular box of enhanced crosslinking in the center of the contact map. In addition, sequences upstream of the blue boundary and downstream of the purple boundary are brought into contact by the head-to-tail pairing of these two boundaries. This generates a third rectangular box of enhanced physical contact in the upper-right corner of the contact map that links sequences in the TAD containing the RhoGEF64C gene to sequences in the TAD containing the internal Mp promoter (black arrowhead). Note that the positioning of the lower-left and upper-right rectangular boxes of enhanced contact in the bin-MP meta-loop is the mirror image of the rectangular boxes of enhanced contact for the CG3294 meta-loop.
Stem-loops versus circle-loops
The results we have reported here demonstrate that boundary:boundary pairing in flies generates loops that can have either a stem-loop or a circle-loop topology. An important question is, what is the relative frequency of stem-loops versus circle-loops in the fly genome? The MicroC contact patterns for the stem-loop and circle-loop versions of the eve TAD are quite distinct. The former is a volcano triangle with a plume while the latter is a volcano triangle flanked by clouds. A survey of the MicroC contact patterns elsewhere in the non-repetitive regions of the fly genome indicates that volcanoes with plumes are rare (~30). For example, there are two volcano triangles with plumes in the Antennapedia complex, and they encompass the deformed and fushi-tarazu genes (Levo et al., 2022). However, since most of the ‘euchromatic’ regions of the fly genome are assembled into TADs whose MicroC profiles resemble that observed for eve circle-loops and the Abd-B region of BX-C, it is possible that much of the fly genome is assembled into circle-loops, not stem-loops.
While this suggestion is consistent with the available data, it is based on contact patterns between neighboring TADs, and important caveats remain. For one, the contact patterns between neighboring TADs can deviate in one way or another from that seen in the Abd-B region. For example, there are TADs in which interactions with one set of neighbors appear to be suppressed as expected for stem-loops, but the classic plume is absent, as interactions are not suppressed with the other neighbors (c.f., Figure 8—figure supplement 1A). In other cases, there is a series of complicated TAD-TAD interactions topped by a rectangular plume (Figure 8—figure supplement 1, purple arrow). For this reason, it will not be possible to draw firm conclusions about the frequency of stem-loops versus circle-loops genome-wide until the relative orientation of the paired boundaries themselves can be determined directly. On the other hand, it is clear from our studies that both classes of TADs must exist in flies. If, as seems likely, a significant fraction of the TADs genome-wide are circle-loops, this would effectively exclude cohesin-based loop extrusion as a general mechanism for TAD formation in flies. In addition, though stem-loops could be generated by a cohesin-dependent mechanism, it is unlikely that this mechanism is operational in flies, as we have shown here and in Bing et al., 2024 that stem-loops in flies are formed by orientation-dependent boundary:boundary pairing.
Another important question is whether our findings have any relevance to the formation and topology of TADs in mammals. In the most common version of the loop extrusion model, the mammalian genome is assembled into an alternating pattern of stem-loops and unanchored loops (Davidson and Peters, 2021; Higashi and Uhlmann, 2022; Perea-Resa et al., 2021). In this case, one might expect to observe volcano triangles topped by plumes alternating with DNA segments that have a considerably lower density of internal contacts. However, this crosslinking pattern is not observed in published MicroC data sets (Hsieh et al., 2020; Krietenstein et al., 2020). Instead of an alternating pattern of high-density TAD triangles separated by regions of low-density contacts, the TAD triangles are generally linked to both neighbors, just as in flies. Moreover, also like in Drosophila, there are few stem-loop volcano TADs topped by plumes. Instead, the crosslinking pattern between neighboring TADs appears similar to that observed for circle-loops in flies. Of course, one problem with these MicroC studies is that the resolution may not be sufficient to detect volcanoes with plumes or the other features predicted by the loop-extrusion model. However, there are no obvious volcanoes with plumes in the much higher resolution RCMC studies of Goel et al., 2023. Instead, the MicroC profiles most closely resemble those seen in the Abd-B region of BX-C (c.f. the Ppm1g locus in Figure 4 of Goel et al., 2023). Moreover, compromising cohesin activity has minimal impact on the TADs in this region of the mouse genome, as evidenced from the MicroC pattern before and after knockdown. Based on these observations, one can reasonably question whether cohesin-mediated loop extrusion is deployed in mammals as the mechanism for not only generating TADs but also determining TAD boundaries. Clearly, validation of the loop-extrusion/CTCF road-block model as currently formulated will require a direct demonstration that mammalian TADs are exclusively either stem-loops or unanchored loops, and that the endpoints are always (or almost always) determined by CTCF roadblocks.
TADs and A/B compartmentalization
A/B compartmentalization has been proposed as a mechanism for subdividing the chromosome into discrete domains that is independent of cohesin-mediated loop extrusion and CTCF. In this model, shared biochemical/biophysical properties that reflect the relative transcriptional state of each chromosomal segment drive block polymer co-segregation into a series of discrete domains (Rowley and Corces, 2018; Rowley et al., 2017). While previous studies suggested that the A and B compartments represented Mb-scale DNA segments, in more recent studies, Harris et al., 2023 found that the average compartment size is on the order of 12 kb. Not only is this much smaller than originally suggested, it is also similar in size to that of most TADs in the Drosophila genome, including the eve TAD. Moreover, in their studies (and also in our data sets), there is close to a one-to-one correspondence between the linear arrangement of individual TADs along the chromosome and the DNA segments that are thought to assemble into discrete domains by co-polymer segregation. This close connection to TADs is also reflected in the patchwork patterns of interacting chromatin domains that are visualized in studies on A/B compartments.
According to this newer version of the compartment model, the chromatin state of each DNA segment determines not only whether it will assemble into a discrete domain, but also how the resulting domain interacts with next-door neighbors, next-next-door neighbors, etc. However, this model does not appear to fit with several of our findings. To begin with, the sequences included in the eve TAD and its patterns of interaction with neighboring TADs are essentially the same in NC14 embryos as they are in 12–16 hr embryos. In the former case, eve is transcriptionally poised (Chen et al., 2013), probably in most or all nuclei, while in the latter case, the entire eve TAD is silenced by a PcG-dependent mechanism in all but a few nuclei (Nègre et al., 2010). However, this transition from potentially active to silenced does not impact the eve TAD, nor does it alter how the eve TAD interacts with the neighboring TADs that are (mostly) transcriptionally active at both stages of development. Similarly, in the meta-loops we have examined, the transcriptional state of the TADs and their contact patterns with their neighbors are not consistent with a strict partitioning of chromosomal segments into one of two compartments. For example, in the CG3294 meta-loop (Figure 8A), the three genes (CG33534, the odorant binding gene Obp22a and the Npc2a gene) that comprise the TAD upstream of the blue boundary would be predicted to be in the same chromatin state; however, while CG33534 and Obp22a are not expressed in embryos, the Npc2a gene is expressed at high levels during embryogenesis and should partition into a separate TAD. The TAD downstream of the blue boundary contains two transcriptionally repressed genes (CG15353 and Nplp4) in 12–16 hr embryos. This TAD physically interacts with the TAD downstream of the purple boundary that contains CG3294 and slf. According to the A/B compartment model, the CG15353 and Nplp4 TAD interacts with the CG3294 and slf TAD because the chromatin in these two TADs share biochemical/biophysical properties that are characteristic of the inactive B compartment. However, unlike CG15353 and Nplp4, both slf and CG3294 are expressed in 12–16 hr embryos, slf at a high level and CG3294 at a low level.
Likewise, if block-polymer co-segregation is the determining factor for both TAD formation and the patterns of TAD:TAD interactions, then our manipulations of the nhomie boundary should have only a minimal impact on the MicroC contact maps, unless there are significant changes in the transcriptional status of eve and its neighbors. In the lambda DNA replacement, the left endpoint of the WT eve TAD (the normal location of nhomie) in 12–16 hr embryos is lost, and instead it appears to map primarily to the right or left boundaries of the TL-3 sub-TAD. TL-3 contains the eIF3j gene, which is expressed at high levels throughout the embryo at this stage, while eve itself is silenced by a PcG-dependent mechanism in all but a small number of cells. The nhomie reverse and homie forward replacements restore the eve TAD. This means that in this instance, TAD formation is mediated by the pairing of the two replacement boundaries with homie (as demonstrated by the viewpoints in Figure 6) and not by partitioning into an A or B compartment. The replacements also alter interactions between eve and the neighboring TADs. Unlike in WT where the eve TAD is physically isolated from its neighbors, the eve TAD interacts with both neighbors in these two replacements. However, the genes in the neighboring TADs do not share the same biochemical/biophysical state—they are active in 12–16 hr embryos, and so should segregate into the A compartment, while eve is inactive and should segregate into the B compartment.
While these observations are inconsistent with a model in which block-polymer co-segregation is responsible for the formation of TADs and determining the pattern of TAD:TAD interactions, this does not rule out a different role, namely in augmenting the insulating activity of boundary elements. One of the defining properties of boundary elements is to restrict the activities of enhancers and silencers, and this helps ensure that the chromatin within a given TAD shares the same biochemical and biophysical properties. The shared biochemical/biophysical properties could in turn enhance the segregation of the chromatin into different compartments, and thus mediate some of the changes we observe. As we cannot rule out the possibility that such biophysical forces augment the functional properties of boundaries, we should add them to the list of downstream events that are dependent upon boundary–boundary interactions and the specific topologies that they can induce. So, while compartment co-segregation may well play a role, on multiple length scales, in mediating the effects of boundaries and other regulatory elements on gene expression and chromosome topology, it certainly cannot ‘replace’ their functional properties as an explanation for those effects.
Materials and methods
Creation of nhomie deletion flies
Request a detailed protocolTo modify nhomie at the eve locus, we used recombinase-mediated cassette exchange (Bateman et al., 2006). First, we inserted two closely positioned attP sites using CRISPR. The donor plasmid for this was constructed as follows. First, a mini-white (mw) gene with Glass binding sites (Fujioka et al., 1999) was inserted into pBlueScript. From the standard mw gene, the Wari insulator (Chetverina et al., 2008) was deleted. Then, two 102 bp attP sequences (Venken et al., 2011) were inserted, one just 5′ of the Glass binding sites and the other at the 3′ end of the modified mw, creating the plasmid pP-attPx2-mw. 5′- and 3′-homologous arms were added to both ends. Two gRNA sequences were cloned into plasmid pCFD4 (Port et al., 2014) (Addgene). The donor and gRNA plasmids were injected into a Cas9 line (y[1] M{vas-Cas9.S}ZH-2A w[1118], Bloomington Drosophila Stock Center). This chromosomal modification resulted in one attP site being inserted in the intron of CG12134, and the other being inserted between the eve 7-stripe enhancer and the 3+7 stripe enhancer. This also deleted 2.2 kb of endogenous sequence, including nhomie and the eve 7-stripe enhancer.
After identifying a successful insertion (NattPmw), mw was replaced by each of the following using RMCE: (1) the previously deleted 2.2 kb, restoring nhomie and the eve 7-stripe enhancer, to create ‘wild-type nhomie’’ (nhomie forward), (2) the same 2.2 kb sequence, but with 600 bp of phage λ DNA in place of 600 bp nhomie (λ DNA), (3) the same 2.2 kb sequence, but with 600 bp nhomie inverted (nhomie reverse), and (4) the same 2.2 kb sequence, but with 600 bp nhomie replaced by a copy of ~600 bp homie in its native orientation in the chromosome (homie forward). Each of these changes was confirmed by sequencing of genomic DNA from the transgenic fly lines.
Analysis of embryonic cuticle patterns and in situ hybridization
Request a detailed protocolTo identify defects in developing embryos, embryos were collected for 2.5 hr, and allowed to develop for an additional 20–21 hr at 25°C. Embryos were dechorionated and mounted in a 1:1 mixture of Hoyer’s medium and lactic acid. Mounted embryos were left at room temperature (RT) until they cleared (12–14 days), and the patterns of ventral abdominal denticles were examined and tallied as follows. Loss of at least one-fifth of a denticle band (in A1-A8) was counted as ‘missing’. Fused denticle bands, which rarely occurred, were also counted as a ‘missing’ band. Minor defects such as those within individual denticle rows were not counted.
Digoxigenin (DIG) in situ hybridization was performed using DIG-labeled anti-sense RNA against CG12134, eIF3j, and TER94. RNA expression was visualized using alkaline phosphatase-conjugated anti-DIG antibody (Roche), using CBIP and NBT as substrates (Roche). Each set of experiments was carried out in parallel to minimize experimental variation. Representative expression patterns are shown in each figure.
HCR-FISH
Request a detailed protocolThe sequences of target genes were obtained from FlyBase (https://www.flybase.org/; Gramates et al., 2022). To design probes, the target gene sequences were submitted to the Molecular Instruments probe design platform (https://molecularinstruments.com/hcr-rnafish; Choi et al., 2016), with parameters set to a 35 probe set size for Drosophila melanogaster. A similar method was designed based on published smFISH methods (Little and Gregor, 2018; Trcek et al., 2017). 100–200 flies were placed in a cage with an apple juice plate at the bottom. For early stages, the embryos were collected for 7 hr, while for later-stage embryos, collections were overnight. Embryos from each plate were washed into collection mesh and dechorionated in bleach for 2 min, then fixed in 5 mL of 4% paraformaldehyde in 1× PBS and 5 mL of heptane for 15 min with horizontal shaking. The paraformaldehyde was then removed and replaced with 5 mL methanol. The embryos were then devitellinized by vortexing for 30 s and washed in 1 mL of methanol twice. Methanol was then removed and replaced by PTw (1× PBS with 0.1% Tween-20) through serial dilution as 7:3, 1:1, and 3:7 methanol:PTw. The embryos were washed twice in 1 mL of PTw and pre-hybridized in 200 μL of probe hybridization buffer for 30 min at 37°C. 0.4 pmol of each probe set were added to the embryos in the probe hybridization buffer, and the embryos were incubated at 37°C for 12–14 hr. The embryos were then washed 3× with probe wash buffer at 37°C for 30 min and 2× with 5× SSCT (5× SSC + 0.1% tween) at RT for 5 min. Then the embryos were pre-amplified with 300 μL amplification buffer for 10 min at 25°C. Meanwhile, 6 pmol of hairpin h1 and h2 were snap-cooled separately (95°C for 90 s, cool to RT with 0.1°C drop per second), and then mixed in 100 μL of amplification buffer at RT. After that, the pre-amplification solution was removed from the embryos, and 100 μL of hairpin h1/h2 mix were added to the embryos. Next, the embryos were incubated for 12–14 hr at RT in the dark. To remove excess hairpins, the embryos were washed in SSCT as follows: 2× for 5 min, 2× for 30 min, and 5× for 5 min. Then, the embryos were washed with 1 mL PTw for 2 min and stained with DAPI/Hoechst at 1 μg/mL for 15 min at RT in the dark. The embryos were then washed with PTw 3× for 5 min. Finally, the embryos were mounted on microscope slides with Vectashield and a #1.5 coverslip for imaging.
Imaging, image analysis, and statistics
Request a detailed protocolEmbryos from HCR-FISH were imaged using a Nikon A1 confocal microscope system, with a Plan Apo ×20/0.75 DIC objective. Z-stack images were taken at interval of 2 μm, 4× average, 1024 × 1024 resolution, and the appropriate laser power and gain were set for 405, 488, 561, and 640 channels to avoid overexposure. Images were processed using ImageJ, and the maximum projection was applied to each of the stack images. To determine the presence of stripes in early embryos, multi-channel images were first split into single channels and the stripe signal was highlighted and detected by the MaxEntropy thresholding method. GraphPad Prism was used for data visualization and statistical analysis. Two-way ANOVA with Tukey’s multiple comparisons test for each pair of groups was used to determine the statistical significance for the percentage of embryos carrying stripes in eIF3j and TER94 channels in each group.
MicroC library construction for the nhomie replacements
Request a detailed protocolEmbryos were collected on yeasted apple juice plates in population cages for 4 hr, incubated for 12 hr at 25°C, then subjected to fixation as follows. Embryos were dechorionated for 2 min in 3% sodium hypochlorite, rinsed with deionized water, and transferred to glass vials containing 5 mL PBST (0.1% Triton-X100 in PBS), 7.5 mL n-heptane, and 1.5 mL fresh 16% formaldehyde. Crosslinking was carried out at RT for exactly 15 min on an orbital shaker at 250 rpm, followed by addition of 3.7 mL 2 M Tris-HCl pH 7.5 and shaking for 5 min to quench the reaction. Embryos were washed twice with 15 mL PBST and subjected to secondary crosslinking. Secondary crosslinking was done in 10 mL of freshly prepared 3 mM final DSG and ESG in PBST for 45 min at RT with passive mixing. The reaction was quenched by addition of 3.7 mL of 2 M Tris-HCl pH7.5 for 5 min, washed twice with PBST, snap-frozen, and stored at –80°C until library construction.
Micro-C libraries were prepared as previously described (Batut et al., 2022) with the following modifications: 50 µL of 12–16 hr embryos were used for each biological replicate. 60U of MNase was used for each reaction to digest chromatin to a mononucleosome:dinucleosome ratio of 4. Libraries were barcoded, pooled, and subjected to paired-end sequencing on an Illumina Novaseq S1 100nt Flowcell (read length 50 bases per mate, 6-base index read).
Two or more independent biological replicates were sequenced for each genotype. For each replicate, >1000 embryos were used, and ~250M reads sequenced. Post-sequencing QC analysis was done for every sample, and the QC reports are available along with the sequence data in GEO (GSE263270). The raw sequencing data are also available in GSE263270 for use in further bioinformatics analysis. The figures present the merged data from all independent biological replicates for each genotype. The total read numbers in the merged data are very similar for each genotype (~500M reads). For NC14 embryo MicroC (Figure 2), public data sets GSE171396 and GSE173518 were used (Batut et al., 2022; Levo et al., 2022).
MicroC data processing
Request a detailed protocolMicroC data for D. melanogaster were aligned to custom genomes edited from the Berkeley Drosophila Genome Project (BDGP) Release 6 reference assembly (dos Santos et al., 2015) with BWA-MEM (Li and Durbin, 2009) using parameters -S -P -5 -M. The resultant BAM files were parsed, sorted, de-duplicated, filtered, and split with Pairtools (https://github.com/open2c/pairtools; Goloborodko, 2024). We removed pairs where only half of the pair could be mapped, or where the MAPQ score was less than three. The resultant files were indexed with Pairix (https://github.com/4dn-dcic/pairix; Lee, 2024). The files from replicates were merged with Pairtools before generating 100 bp contact matrices using Cooler (Abdennur and Mirny, 2020). Finally, balancing and Mcool file generation were performed with Cooler’s Zoomify tool.
Virtual 4C profiles were extracted from individual replicates using FAN-C (Kruse et al., 2020) at 400 bp resolution. The values were summed across replicates and smoothed across three bins (1.2 kb). The homie viewpoint was set to the 549nt homie sequence that was defined in previous studies (Fujioka et al., 2016; Fujioka et al., 2009).
Appendix 1
Reagent type (species) or resource | Designation | Source or reference | Identifiers | Additional information |
---|---|---|---|---|
Gene (Drosophila melanogaster) | eve | FlyBase | FBgn0000606 | |
Gene (D. melanogaster) | CG12134 | FlyBase | FBgn0033471 | |
Gene (D. melanogaster) | eIF3j | FlyBase | FBgn0027619 | |
Gene (D. melanogaster) | TER94 | FlyBase | FBgn0286784 | |
Genetic reagent (D. melanogaster) | y1 M{vas-Cas9}ZH-2A w1118/FM7c | Bloomington Drosophila Stock Center | 51323 | |
Recombinant DNA reagent (plasmid) | pCFD4-U6:1_U6:3tandemgRNAs | Addgene | 49411 | |
Chemical compound, drug | n-Heptane | Fisher Chemical | O3008-4 | |
Chemical compound, drug | Paraformaldehyde 20% solution, EM Grade | Electron Microscopy Sciences | 15713S | |
Chemical compound, drug | Formaldehyde, 16%, methanol free, Ultra Pure | Polysciences Inc | 18814-10 | |
Chemical compound, drug | PBS – phosphate-buffered saline (10×) pH 7.4, RNase-free | Thermo Fisher | AM9624 | |
Chemical compound, drug | Tween 20 | Sigma | P1379 | |
Chemical compound, drug | Triton X-100 | Bio-Rad | 161-0407 | |
Chemical compound, drug | Tris base | Sigma | 11814273001 | |
Chemical compound, drug | Methanol | Fisher Chemical | 203403 | |
Chemical compound, drug | SSC, 20× | Thermo Fisher | 15557044 | |
Chemical compound, drug | Formamide | Thermo Fisher | 17899 | |
Chemical compound, drug | Dextran sulfate | Sigma | D8906 | |
Chemical compound, drug | Salmon Sperm DNA | Thermo Fisher | AM9680 | |
Chemical compound, drug | Ribonucleoside Vanadyl Complex | NEB | S1402S | |
Chemical compound, drug | Nuclease-free BSA | Sigma | 126609 | |
Chemical compound, drug | Triethylammonium acetate | Sigma | 625718 | |
Chemical compound, drug | dGTP (100 MM) | VWR | 76510-208 | |
Chemical compound, drug | dTTP (100 MM) | VWR | 76510-224 | |
Chemical compound, drug | Lonza NuSieve 3:1 Agarose | Thermo Fisher | BMA50090 | |
Other | T4 DNA ligase | NEB | M0202L | Enzyme |
Chemical compound, drug | Biotin-11-dCTP | Jena Bioscience | NU-809-BIOX | |
Chemical compound, drug | Biotin-14-dATP | Jena Bioscience | NU-835-BIO14 | |
Commercial assay or kit | Qubit dsDNA HS Assay Kit | Life Technologies Corp. | Q32851 | |
Chemical compound, drug | Atto 633 NHS ester | Sigma | 01464 | |
Chemical compound, drug | Phase Lock Gel, QuantaBio - 2302830, Phase Lock Gel Heavy | VMR | 10847-802 | |
Commercial assay or kit | NEBNext Ultra II DNA Library Prep Kit for Illumina | NEB | E7645S | |
Commercial assay or kit | Ampure Xp 5 ml Kit | Thermo Fisher | NC9959336 | |
Commercial assay or kit | Hifi Hotstart Ready Mix | Thermo Fisher | 501965217 | |
Commercial assay or kit | Dynabeads MyOne Streptavidin C1 | Life Technologies Corp. | 65001 | |
Chemical compound, drug | cOmplete, EDTA-free Protease Inhibitor Cocktail | Sigma | 11873580001 | |
Chemical compound, drug | N,N-Dimethylformamide | Sigma | 227056 | |
Chemical compound, drug | Potassium acetate solution | Sigma | 95843 | |
Chemical compound, drug | DSG (disuccinimidyl glutarate) | Thermo Fisher | PI20593 | |
Other | T4 Polynucleotide Kinase – 500 units | NEB | M0201S | Enzyme |
Other | DNA Polymerase I, Large (Klenow) Fragment – 1000 units | NEB | M0210L | Enzyme |
Commercial assay or kit | End-it DNA End Repair Kit | Thermo Fisher | NC0105678 | |
Other | Proteinase K recomb. 100 mg | Sigma | 3115879001 | Enzyme |
Other | Nuclease Micrococcal (s7) | Thermo Fisher | NC9391488 | Enzyme |
Chemical compound, drug | EGS (ethylene glycol bis(succinimidyl succinate)) | Thermo Fisher | PI21565 | |
Commercial assay or kit | Atto 565 NHS ester | Sigma | 72464 | |
Commercial assay or kit | HCR RNA-FISH Custom Probe Set: eve | Molecular Instruments | Custom probes | |
Commercial assay or kit | HCR RNA-FISH Custom Probe Set: ter94 | Molecular Instruments | Custom probes | |
Commercial assay or kit | HCR RNA-FISH Custom Probe Set: CG12134 | Molecular Instruments | Custom probes | |
Commercial assay or kit | HCR RNA-FISH Custom Probe Set: eIF3j | Molecular Instruments | Custom probes | |
Commercial assay or kit | HCR Amplifier B1, 488 | Molecular Instruments | Custom probes | |
Commercial assay or kit | HCR Amplifier B2, 564 | Molecular Instruments | Custom probes | |
Commercial assay or kit | HCR Amplifier B3, 647 | Molecular Instruments | Custom probes | |
Commercial assay or kit | HCR Buffers | Molecular Instruments | Custom probes | |
Commercial assay or kit | NEBNext Multiplex Oligos for Illumina | NEB | E7335S | |
Software, algorithm | Fiji (ImageJ) | Schindelin et al., 2012 | fiji.sc | |
Software, algorithm | NIS element | Nikon | microscope.healthcare.nikon.com/products/software/nis-elements | |
Software, algorithm | GraphPad Prism 8 | GraphPad Software | https://www.graphpad.com/ | |
Software, algorithm | HiGlass | Kerpedjiev et al., 2018 | https://higlass.io/app | |
Software, algorithm | bwa | Li and Durbin, 2009 | https://bio-bwa.sourceforge.net/ | |
Software, algorithm | samtools | GitHub/open source | https://samtools.github.io | |
Software, algorithm | pairsamtools | Goloborodko et al., 2024 | https://github.com/mirnylab/pairsamtools | |
Software, algorithm | pairix | Lee, 2024 | https://github.com/4dn-dcic/pairix | |
Software, algorithm | cooler | Abdennur and Mirny, 2020; Abdennur, 2016 | https://github.com/open2c/cooler | |
Software, algorithm | Miniconda | Anaconda | https;//docs.conda.io/en/latest/miniconda/ | |
Software, algorithm | Snakemake | GitHub/open source | https://snakemake.github.io |
Data availability
Sequence data are available at GEO GSE263270. Confocal images are available on Open Science Framework at https://doi.org/10.17605/OSF.IO/6PYBM.
-
NCBI Gene Expression OmnibusID GSE263270. Chromosome Structure II: Stem-loops and circle-loops.
-
Open Science FrameworkID 6pybm/. Stem-loop and circle-loop TADs generated by directional pairing of boundary elements have distinct physical and regulatory properties.
-
NCBI Gene Expression OmnibusID GSE171396. Genome organization controls transcriptional dynamics during development.
-
NCBI Gene Expression OmnibusID GSE173518. Transcriptional coupling of distant regulatory genes in living embryos.
References
-
To loop or not to loop: what is the role of TADs in enhancer function and gene regulation?Current Opinion in Genetics & Development 67:119–129.https://doi.org/10.1016/j.gde.2020.12.015
-
Dynamic interplay between enhancer-promoter topology and gene activityNature Genetics 50:1296–1303.https://doi.org/10.1038/s41588-018-0175-z
-
Mapping a multiplexed zoo of mRNA expressionDevelopment 143:3632–3637.https://doi.org/10.1242/dev.140137
-
Genome folding through loop extrusion by SMC complexesNature Reviews. Molecular Cell Biology 22:445–464.https://doi.org/10.1038/s41580-021-00349-7
-
Successive gain of insulator proteins in arthropod evolutionEvolution; International Journal of Organic Evolution 67:2945–2956.https://doi.org/10.1111/evo.12155
-
New tools in the box: an evolutionary synopsis of chromatin insulatorsTrends in Genetics 30:161–171.https://doi.org/10.1016/j.tig.2014.03.004
-
SMC complexes: Lifting the lid on loop extrusionCurrent Opinion in Cell Biology 74:13–22.https://doi.org/10.1016/j.ceb.2021.12.003
-
Higher-order chromosomal structures mediate genome functionJournal of Molecular Biology 432:676–681.https://doi.org/10.1016/j.jmb.2019.10.014
-
A group of scs elements function as domain boundaries in an enhancer-blocking assayMolecular and Cellular Biology 12:2424–2431.https://doi.org/10.1128/mcb.12.5.2424-2431.1992
-
Functional interaction between the Fab-7 and Fab-8 boundaries and the upstream promoter region in the Drosophila Abd-B geneMolecular and Cellular Biology 28:4188–4195.https://doi.org/10.1128/MCB.00229-08
-
Single mRNA molecule detection in DrosophilaMethods in Molecular Biology 1649:127–142.https://doi.org/10.1007/978-1-4939-7213-5_8
-
Cohesin: behind dynamic genome topology and gene expression reprogrammingTrends in Cell Biology 31:760–773.https://doi.org/10.1016/j.tcb.2021.03.005
-
Organizational principles of 3D genome architectureNature Reviews. Genetics 19:789–800.https://doi.org/10.1038/s41576-018-0060-8
-
Fiji: an open-source platform for biological-image analysisNature Methods 9:676–682.https://doi.org/10.1038/nmeth.2019
-
mRNA quantification using single-molecule FISH in Drosophila embryosNature Protocols 12:1326–1348.https://doi.org/10.1038/nprot.2017.030
-
Genetic and molecular analysis of chromatin domainsCold Spring Harbor Symposia on Quantitative Biology 58:45–54.https://doi.org/10.1101/sqb.1993.058.01.008
-
The Mcp element mediates stable long-range chromosome-chromosome interactions in DrosophilaMolecular Biology of the Cell 17:2158–2165.https://doi.org/10.1091/mbc.e06-01-0049
Article and author information
Author details
Funding
New Jersey Commission on Cancer Research (COCR23PDF011)
- Wenfan Ke
Histochemical Society (Keystone Grant)
- Wenfan Ke
National Institute of General Medical Sciences (R35 GM126975)
- Paul Schedl
National Institute of General Medical Sciences (R01 GM137062)
- James B Jaynes
The funders had no role in study design, data collection and interpretation, or the decision to submit the work for publication.
Acknowledgements
We thank Gordon Grey for running the fly food facility at Princeton, members of the Lewis Sigler Genomics Core facility for their invaluable assistance with DNA sequencing, and Qing Liu for excellent technical assistance. We would also like to thank members of MOL431 for creative input. Special thanks to Olga Kyrchanova, Daria Chetverina, Maksim Erokhin, Pavel Georigev, Tsutomu Aoki, Girish Deshpande, Airat Ibragimov, Sergey Ryabichko, Yuri Pritykin, Alex Ostrin, Xinyang Bing, Xiao Li, and Mike Levine for stimulating discussions and sharing unpublished data.
Version history
- Sent for peer review:
- Preprint posted:
- Reviewed Preprint version 1:
- Reviewed Preprint version 2:
- Version of Record published:
Cite all versions
You can cite all versions using the DOI https://doi.org/10.7554/eLife.94114. This DOI represents all versions, and will always resolve to the latest one.
Copyright
© 2024, Ke, Fujioka et al.
This article is distributed under the terms of the Creative Commons Attribution License, which permits unrestricted use and redistribution provided that the original author and source are credited.
Metrics
-
- 1,153
- views
-
- 84
- downloads
-
- 10
- citations
Views, downloads and citations are aggregated across all versions of this paper published by eLife.
Download links
Downloads (link to download the article as PDF)
Open citations (links to open the citations from this article in various online reference manager services)
Cite this article (links to download the citations from this article in formats compatible with various reference manager tools)
Further reading
-
- Chromosomes and Gene Expression
RNA interference (RNAi) is a conserved pathway that utilizes Argonaute proteins and their associated small RNAs to exert gene regulatory function on complementary transcripts. While the majority of germline-expressed RNAi proteins reside in perinuclear germ granules, it is unknown whether and how RNAi pathways are spatially organized in other cell types. Here, we find that the small RNA biogenesis machinery is spatially and temporally organized during Caenorhabditis elegans embryogenesis. Specifically, the RNAi factor, SIMR-1, forms visible concentrates during mid-embryogenesis that contain an RNA-dependent RNA polymerase, a poly-UG polymerase, and the unloaded nuclear Argonaute protein, NRDE-3. Curiously, coincident with the appearance of the SIMR granules, the small RNAs bound to NRDE-3 switch from predominantly CSR-class 22G-RNAs to ERGO-dependent 22G-RNAs. NRDE-3 binds ERGO-dependent 22G-RNAs in the somatic cells of larvae and adults to silence ERGO-target genes; here we further demonstrate that NRDE-3-bound, CSR-class 22G-RNAs repress transcription in oocytes. Thus, our study defines two separable roles for NRDE-3, targeting germline-expressed genes during oogenesis to promote global transcriptional repression, and switching during embryogenesis to repress recently duplicated genes and retrotransposons in somatic cells, highlighting the plasticity of Argonaute proteins and the need for more precise temporal characterization of Argonaute-small RNA interactions.
-
- Chromosomes and Gene Expression
- Genetics and Genomics
A new method for mapping torsion provides insights into the ways that the genome responds to the torsion generated by RNA polymerase II.