Zn2+ is essential for Ca2+ oscillations in mouse eggs
eLife assessment
This article reports an important series of results showing the relationship between oscillatory zinc and calcium fluctuations during egg activation and fertilization. Compelling evidence using several complimentary approaches provides further insight into the signals for proper egg activation that underpin successful fertilization and embryo development. The findings are significant because they may lead to improvements in assisted reproduction methods.
https://doi.org/10.7554/eLife.88082.3.sa0Important: Findings that have theoretical or practical implications beyond a single subfield
- Landmark
- Fundamental
- Important
- Valuable
- Useful
Compelling: Evidence that features methods, data and analyses more rigorous than the current state-of-the-art
- Exceptional
- Compelling
- Convincing
- Solid
- Incomplete
- Inadequate
During the peer-review process the editor and reviewers write an eLife Assessment that summarises the significance of the findings reported in the article (on a scale ranging from landmark to useful) and the strength of the evidence (on a scale ranging from exceptional to inadequate). Learn more about eLife Assessments
Abstract
Changes in the intracellular concentration of free calcium (Ca2+) underpin egg activation and initiation of development in animals and plants. In mammals, the Ca2+ release is periodical, known as Ca2+ oscillations, and mediated by the type 1 inositol 1,4,5-trisphosphate receptor (IP3R1). Another divalent cation, zinc (Zn2+), increases exponentially during oocyte maturation and is vital for meiotic transitions, arrests, and polyspermy prevention. It is unknown if these pivotal cations interplay during fertilization. Here, using mouse eggs, we showed that basal concentrations of labile Zn2+ are indispensable for sperm-initiated Ca2+ oscillations because Zn2+-deficient conditions induced by cell-permeable chelators abrogated Ca2+ responses evoked by fertilization and other physiological and pharmacological agonists. We also found that chemically or genetically generated eggs with lower levels of labile Zn2+ displayed reduced IP3R1 sensitivity and diminished ER Ca2+ leak despite the stable content of the stores and IP3R1 mass. Resupplying Zn2+ restarted Ca2+ oscillations, but excessive Zn2+ prevented and terminated them, hindering IP3R1 responsiveness. The findings suggest that a window of Zn2+ concentrations is required for Ca2+ responses and IP3R1 function in eggs, ensuring optimal response to fertilization and egg activation.
Introduction
Vertebrate eggs are arrested at the metaphase stage of the second meiosis (MII) when ovulated because they have an active Cdk1/cyclin B complex and inactive APC/CCdc20 (Heim et al., 2018). Release from MII initiates egg activation, the first hallmark of embryonic development (Ducibella et al., 2002; Schultz and Kopf, 1995). The universal signal of egg activation is an increase in the intracellular concentration of calcium (Ca2+) (Ridgway et al., 1977; Stricker, 1999). Ca2+ release causes the inactivation of the APC/C inhibitor Emi2, which enhances cyclin B degradation and induces meiotic exit (Lorca et al., 1993; Shoji et al., 2006; Suzuki et al., 2010a). In mammals, the stereotypical fertilization Ca2+ signal, oscillations, consists of transient but periodical Ca2+ increases that promote progression into interphase (Deguchi et al., 2000; Miyazaki et al., 1986). The sperm-borne phospholipase C zeta1 (PLCζ) persistently stimulates the production of inositol 1,4,5-trisphosphate (IP3) (Matsu-ura et al., 2019; Saunders et al., 2002; Wu et al., 2001) that binds its cognate receptor in the endoplasmic reticulum (ER), IP3R1, and causes Ca2+ release from the egg’s main Ca2+ reservoir (Wakai et al., 2019). The intake of extracellular Ca2+ via plasma membrane channels and transporters ensures the persistence of the oscillations (Miao et al., 2012; Stein et al., 2020; Wakai et al., 2019; Wakai et al., 2013).
Before fertilization, maturing oocytes undergo cellular and biochemical modifications (see for review Ajduk et al., 2008). The nucleus of immature oocytes, known as the germinal vesicle (GV), undergoes the breakdown of its envelope, marking the onset of maturation and setting in motion a series of cellular events that culminate with the release of the first polar body, the correct ploidy for fertilization, and re-arrest at MII (Eppig, 1996). Other organelles are also reorganized, such as cortical granules migrate to the cortex for exocytosis and polyspermy block, mitochondria undergo repositioning, and the cytoplasm’s redox state becomes progressively reduced to promote the exchange of the sperm’s protamine load (Liu, 2011; Perreault et al., 1988; Wakai et al., 2014). Wide-ranging adaptations also occur in the Ca2+ release machinery to produce timely and protracted Ca2+ oscillations following sperm entry (Fujiwara et al., 1993; Lawrence et al., 1998), including the increase in the content of the Ca2+ stores, ER reorganization with cortical cluster formation, and increased IP3R1 sensitivity (Lee et al., 2006; Wakai et al., 2012). The total intracellular levels of zinc (Zn2+) also remarkably increase during maturation, amounting to a 50% rise, which is necessary for oocytes to proceed to the telophase I of meiosis and beyond (Kim et al., 2010). Notably, after fertilization, Zn2+ levels need to decrease, as Emi2 is a Zn2+-associated molecule, and high Zn2 levels prevent MII exit (Bernhardt et al., 2012; Shoji et al., 2014; Suzuki et al., 2010b). Following the initiation of Ca2+ oscillations, approximately 10–20% of the Zn2+ accrued during maturation is ejected during the Zn2+ sparks, a conserved event in vertebrates and invertebrate species (Converse and Thomas, 2020; Kim et al., 2011; Mendoza et al., 2022; Que et al., 2019; Seeler et al., 2021; Tokuhiro and Dean, 2018; Wozniak et al., 2020; Zhang et al., 2016). The use of Zn2+ chelators such as N,N,N,N-tetrakis (2-pyridinylmethyl)–1,2-ethylenediamine (TPEN) to create Zn2+-deficient conditions buttressed the importance of Zn2+ during meiotic transitions (Kim et al., 2010; Suzuki et al., 2010b). However, whether the analogous dynamics of Ca2+ and Zn2+ during maturation imply crosstalk and Zn2+ levels modulate Ca2+ release during fertilization is unknown.
IP3Rs are the most abundant intracellular Ca2+ release channel in non-muscle cells (Berridge, 2016). They form a channel by assembling into tetramers with each subunit of ~270 kDa MW (Taylor and Tovey, 2010). Mammalian eggs express the type I IP3R, the most widespread isoform (Fissore et al., 1999; Parrington et al., 1998). IP3R1 is essential for egg activation because its inhibition precludes Ca2+ oscillations (Miyazaki and Ito, 2006; Miyazaki et al., 1992; Xu et al., 2003). Myriad and occasionally cell-specific factors influence Ca2+ release through the IP3R1 (Taylor and Tovey, 2010). For example, following fertilization, IP3R1 undergoes ligand-induced degradation caused by the sperm-initiated long-lasting production of IP3 that effectively reduces the IP3R1 mass (Brind et al., 2000; Jellerette et al., 2000). Another regulatory mechanism is Ca2+, a universal cofactor, which biphasically regulates IP3Rs’ channel opening (Iino, 1990; Jean and Klee, 1986), congruent with several Ca2+ and calmodulin binding sites on the channel’s sequence (Sienaert et al., 1997; Sipma et al., 1999). Notably, Zn2+ may also participate in IP3R1 regulation. Recent studies using electron cryomicroscopy (cryoEM), a technique that allows peering into the structure of IP3R1 with a near-atomic resolution, have revealed that a helical linker (LNK) domain near the C-terminus mediates the coupling between the N- and C-terminal ends necessary for channel opening (Fan et al., 2015). The LNK domain contains a putative zinc-finger motif proposed to be vital for IP3R1 function (Fan et al., 2015; Paknejad and Hite, 2018). Therefore, the exponential increase in Zn2+ levels in maturing oocytes, besides its essential role in meiosis progression, may optimize the IP3R1 function, revealing hitherto unknown cooperation between these cations during fertilization.
Here, we examined whether crosstalk between Ca2+ and Zn2+ is required to initiate and sustain Ca2+ oscillations and maintain Ca2+ store content in MII eggs. We found that Zn2+-deficient conditions inhibited Ca2+ release and oscillations without reducing Ca2+ stores, IP3 production, IP3R1 expression, or altering the viability of eggs or zygotes. We show instead that Zn2+ deficiency impaired IP3R1 function and lessened the receptor’s ability to gate Ca2+ release out of the ER. Remarkably, resupplying Zn2+ re-established the oscillations interrupted by low Zn2+, although persistent increases in intracellular Zn2+ were harmful, disrupting the Ca2+ responses and preventing egg activation. Together, the results show that besides contributing to oocyte maturation, Zn2+ has a central function in Ca2+ homeostasis such that optimal Zn2+ concentrations ensure IP3R1 function and the Ca2+ oscillations required for initiating embryo development.
Results
TPEN dose-dependently lowers intracellular Zn2+ and inhibits sperm-initiated Ca2+ oscillations
TPEN is a cell-permeable, non-specific chelator with a high affinity for transition metals widely used to study their function in cell physiology (Arslan et al., 1985; Lo et al., 2020). Mouse oocytes and eggs have exceedingly high intracellular concentrations of Zn2+ (Kim et al., 2011; Kim et al., 2010), and the TPEN-induced defects in the progression of meiosis have been ascribed to its chelation (Bernhardt et al., 2011; Kim et al., 2010). In support of this view, the Zn2+ levels of cells showed acute reduction after TPEN addition, as reported by indicators such as FluoZin-3 (Arslan et al., 1985; Gee et al., 2002; Suzuki et al., 2010b). Studies in mouse eggs also showed that the addition of low µM (40–100) concentrations of TPEN disrupted Ca2+ oscillations initiated by fertilization or SrCl2 (Lawrence et al., 1998; Suzuki et al., 2010b), but the mechanism(s) and target(s) of the inhibition remained unknown. To gain insight into this phenomenon, we first performed dose-titration studies to determine the effectiveness of TPEN in lowering Zn2+ in eggs. The addition of 2.5 µM TPEN protractedly reduced Zn2+ levels, whereas 5 and 10 µM TPEN acutely and persistently reduced FluoZin-3 fluorescence (Figure 1A). These concentrations of TPEN are higher than the reported free Zn2+ concentrations in cells, but within range of those found in typical culture conditions (Lo et al., 2020; Qin et al., 2011). We next determined the concentrations of TPEN required to abrogate fertilization-initiated oscillations. Following intracytoplasmic sperm injection (ICSI), we monitored Ca2+ responses while increasing TPEN concentrations. As shown in Figure 1B, 5 and 10 µM TPEN effectively blocked ICSI-induced Ca2+ oscillations in over half of the treated cells, and the remaining eggs, after a prolonged interval, resumed lower-frequency rises (Figure 1B, center panels). Finally, 50 µM or greater concentrations of TPEN permanently blocked these oscillations (Figure 1B, right panel). It is noteworthy that at the time of addition TPEN concentrations of 5 µM or above induce a sharp drop in basal Fura-2 F340/F380 ratios, consistent with Fura-2’s high affinity for Zn2+ (Snitsarev et al., 1996).
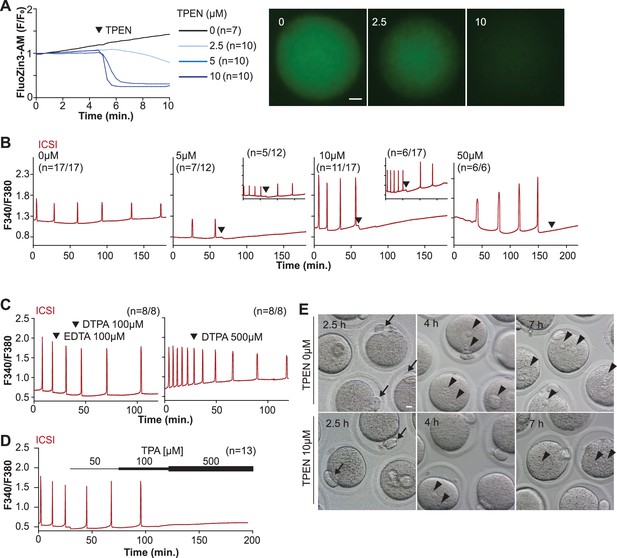
TPEN-induced Zn2+ deficiency inhibits fertilization-initiated Ca2+ oscillations in a dose-dependent manner.
(A) Left panel: representative normalized Zn2+ recordings of MII eggs loaded with FluoZin-3AM following the addition of increasing concentrations of TPEN (0 µM, DMSO, black trace; 2.5 µM, sky blue; 5 µM, blue; 10 µM, navy). TPEN was directly added to the monitoring media. Right panel: representative fluorescent images of MII eggs loaded FluoZin-3AM supplemented with 0, 2.5, and 10 µM of TPEN. Scale bar: 10 µm. (B–D) (B) Representative Ca2+ oscillations following intracytoplasmic sperm injection (ICSI) after the addition of 0, 5, 10, or 50 µM TPEN (arrowheads). Insets show representative traces for eggs that resumed Ca2+ oscillations after TPEN. (C) As above, but following the addition of 100 µM EDTA, 100 or 500 µM DTPA (time of addition denoted by arrowheads). (D) Ca2+ oscillations following ICSI after the addition of 50, 100, and 500 µM TPA (horizontal bars of increasing thickness). (E) Representative bright field images of ICSI-fertilized eggs 2.5, 4, and 7 hr after sperm injection. Arrows and arrowheads denote the second polar body and pronuclear (PN) formation, respectively. Scale bar: 10 µm.
We next used membrane-permeable and -impermeable chelators to assess whether TPEN inhibited Ca2+ oscillations by chelating Zn2+ from intracellular or extracellular compartments. The addition of the high-affinity but cell-impermeable Zn2+ chelators DTPA and EDTA neither terminated nor temporarily interrupted ICSI-induced Ca2+ oscillations (Figure 1C), although protractedly slowed them down, possibly because of chelation and lowering of external Ca2+ (Figure 1C). These results suggest that chelation of external Zn2+ does not affect the continuation of oscillations. We cannot determine that EDTA successfully chelated all external Zn2+, but the evidence that the addition of EDTA to the monitoring media containing cell-impermeable FluoZin-3 caused a marked reduction in fluorescence suggests that a noticeable fraction of the available Zn2+ was sequestered (Figure 1—figure supplement 1). Similarly, injection of mPlcz1 mRNA in eggs incubated in Ca2+ and Mg2+-free media supplemented with EDTA, to maximize the chances of chelation of external Zn2+, initiated low-frequency but persistent oscillations, and addition of Ca2+ and Mg2+ restored the physiological periodicity (Figure 1—figure supplement 1). Lastly, another Zn2+-permeable chelator, TPA, blocked the ICSI-initiated Ca2+ oscillations but required higher concentrations than TPEN (Figure 1D). Collectively, the data suggest that basal levels of labile internal Zn2+ are essential to sustain the fertilization-initiated Ca2+ oscillations in eggs.
We next evaluated whether Zn2+ depletion prevented the completion of meiosis and pronuclear (PN) formation. To this end, ICSI-fertilized eggs were cultured in the presence of 10 μM TPEN for 8 hr, during which the events of egg activation were examined (Figure 1E and Table 1). All fertilized eggs promptly extruded second polar bodies regardless of treatment (Figure 1E). TPEN, however, impaired PN formation, and by 4 or 7 hr post-ICSI, most treated eggs failed to show PNs, unlike controls (Figure 1E and Table 1). Together, these results demonstrate that depletion of Zn2+ terminates Ca2+ oscillations and delays or prevents events of egg activation, including PN formation.
Addition of TPEN after intracytoplasmic sperm injection (ICSI) does not prevent extrusion of the second polar body but precludes pronuclear (PN) formation.
Group* | No. of zygotes | Second polar body (2.5 hr) | PN | |
---|---|---|---|---|
4 hr | 7 hr | |||
Untreated | 26 | 25 (96.1%) | 23 (88.5%) | 23 (88.5%) |
TPEN (10 µM) | 27 | 24 (88.9%) | 1 (3.7%)*** | 2 (7.4%)*** |
-
***p<0.001.
-
*
Data from three different replicates for each group.
TPEN is a universal inhibitor of Ca2+ oscillations in eggs
Mammalian eggs initiate Ca2+ oscillations in response to numerous stimuli and conditions (Miyazaki and Ito, 2006; Wakai and Fissore, 2013). Fertilization and its release of PLCζ stimulate the phosphoinositide pathway, producing IP3 and Ca2+ oscillations (Miyazaki, 1988; Saunders et al., 2002). Neurotransmitters such as acetylcholine (Ach) and other G-protein-coupled receptor agonists engage a similar mechanism (Dupont et al., 1996; Kang et al., 2003), although in these cases, IP3 production occurs at the plasma membrane and is short-lived (Kang et al., 2003; Swann and Parrington, 1999). Agonists such as SrCl2 and thimerosal generate oscillations by sensitizing IP3R1 without producing IP3. The mechanism(s) of SrCl2 is unclear, although its actions are reportedly directly on the IP3R1 (Hajnóczky and Thomas, 1997; Hamada et al., 2003; Nomikos et al., 2015; Nomikos et al., 2011; Sanders et al., 2018). Thimerosal oxidizes dozens of thiol groups in the receptor, which enhances the receptor’s sensitivity and ability to release Ca2+ (Bootman et al., 1992; Evellin et al., 2002; Joseph et al., 2018). We took advantage of the varied points at which the mentioned agonists engage the phosphoinositide pathway to examine TPEN’s effectiveness in inhibiting their effects. mPlcz1 mRNA injection, like fertilization, induces persistent Ca2+ oscillations, although mPlcz1’s tends to be more robust. Consistent with this, the addition of 10 and 25 µM TPEN transiently interrupted or belatedly terminated oscillations, whereas 50 µM acutely stopped all responses (Figure 2A). By contrast, SrCl2-initiated rises were the most sensitive to Zn2+-deficient conditions, with 2.5 µM TPEN nearly terminating all oscillations that 5 µM did (Figure 2B). TPEN was equally effective in ending the Ach-induced Ca2+ responses (Figure 2C), but curbing thimerosal responses required higher concentrations (Figure 2D). Lastly, we ruled out that downregulation of IP3R1 was responsible for the slow-down or termination of the oscillations by TPEN. To accomplish this, we examined the IP3R1 mass in eggs (Jellerette et al., 2004) with and without TPEN supplementation and injection of mPlcz1 mRNA. By 4 hr post-injection, Plcz1 induced the expected downregulation of IP3R1 reactivity, whereas it was insignificant in TPEN-treated and Plcz1 mRNA-injected eggs, as it was in uninjected control eggs (Figure 2F). These findings together show that Zn2+ deficiency inhibits the IP3R1-mediated Ca2+ oscillations independently of IP3 production or loss of receptor, suggesting a role of Zn2+ on IP3R1 function (Figure 2E).
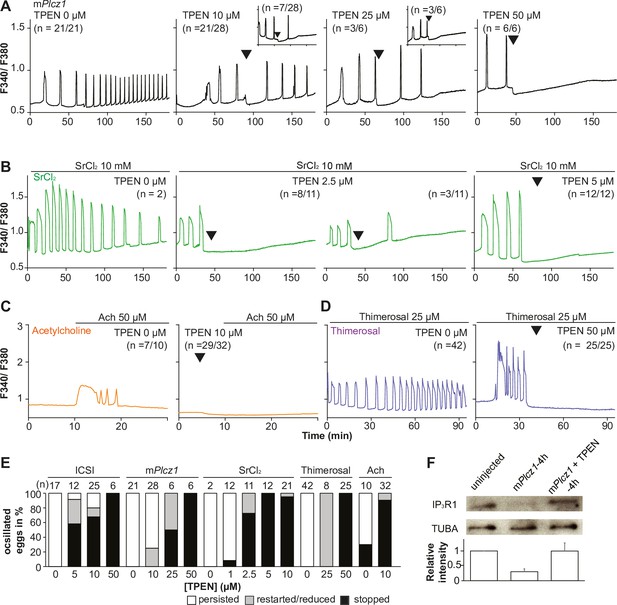
TPEN dose-dependently inhibits Ca2+ oscillations in eggs triggered by a broad spectrum of agonists that stimulate the PI pathway or IP3R1.
(A–D) Representative Ca2+ responses induced by (A) mPlcz1 mRNA microinjection (0.01 µg/µl, black traces), (B) strontium chloride (10 mM, green), (C) acetylcholine chloride (50 µM, orange), and (D) thimerosal (25 µM, purple) in MII eggs. Increasing concentrations of TPEN were added to the monitoring media (arrowheads above traces denotes the time of adding). Insets in the upper row show representative traces of eggs that stop oscillating despite others continuing to oscillate. (E) Each bar graph summarizes the TPEN effect on Ca2+ oscillations at the selected concentrations for each of the agonists in (A–D). (F) Western blot showing the intensities of IP3R1 and alpha-tubulin bands in MII eggs or in eggs injected with mPlcz1 mRNA and incubated or not with TPEN above (p<0.01). Thirty eggs per lane in all cases. This experiment was repeated twice, and the mean relative intensity of each blot is shown in the bar graph below.
-
Figure 2—source data 1
IP3R1 and TUBA western blottings.
- https://cdn.elifesciences.org/articles/88082/elife-88082-fig2-data1-v1.zip
Zn2+ depletion reduces IP3R1-mediated Ca2+ release
To directly assess the inhibitory effects of TPEN on IP3R1 function, we used caged IP3 (cIP3) that, after short UV pulses, releases IP3 into the ooplasm (Wakai et al., 2012; Walker et al., 1987). To exclude the possible contribution of external Ca2+ to the responses, we performed the experiments in Ca2+-free media. In response to sequential cIP3 release 5 min apart, control eggs displayed corresponding Ca2+ rises that occasionally transitioned into short-lived oscillations (Figure 3A). The addition of TPEN after the third cIP3 release prevented the subsequent Ca2+ response and prematurely terminated the in-progress Ca2+ rises (Figure 3B, inset). Pre-incubation of eggs with TPEN precluded cIP3-induced Ca2+ release, even after 5 s UV exposure (Figure 3C). The addition of excess ZnSO4 (100 µM) overcame TPEN’s inhibitory effects only if added before (Figure 3E) and not after the addition of TPEN (Figure 3D). Similar concentrations of MgCl2 or CaCl2 failed to reverse TPEN effects (Figure 3F and G). Together, the results show that Zn2+ is required for IP3R1-mediated Ca2+ release downstream of IP3 production, appearing to interfere with receptor gating, as suggested by TPEN’s rapid termination of in-progress Ca2+ rises and ongoing oscillations.
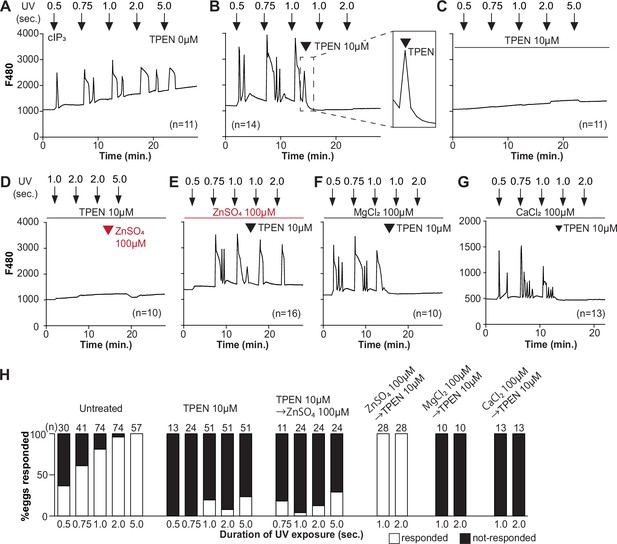
TPEN inhibition of cIP3-induced Ca2+ release is precluded by ZnSO4 supplementation before TPEN exposure.
(A–G) Representative Ca2+ responses in MII eggs triggered by the release of caged IP3 (cIP3) induced by UV light pulses of increasing duration (arrows). (A) A representative control trace without TPEN, and (B) following the addition of 10 µM TPEN between the third and the fourth pulses. The broken line rectangle is magnified in the inset, farthest right side of the panel displaying the near immediate termination of an ongoing rise. (C, D) Recordings started in the presence of 10 µM TPEN but in (D) 100 µM ZnSO4 was added between the second and the third pulses. (E) Recording started in the presence of 100 µM ZnSO4 followed by the addition of 10 µM TPEN between the third and the fourth pulses. (F, G) Recording started in the presence of 100 µM MgSO4 (F) or 100 µM CaCl2 (G) and 10 µM TPEN added as above. Arrowheads above the different panels indicate the time of TPEN or divalent cation addition. (H) Bar graphs summarizing the number and percentages of eggs that responded to a given duration of UV pulses under each of the TPEN ± divalent ions.
ERp44 is an ER luminal protein of the thioredoxin family that interacts with the IP3R1, reportedly inhibiting its ability to mediate Ca2+ release (Higo et al., 2005). The localization of ERp44 in the ER-Golgi intermediate compartment of somatic cells correlates with Zn2+’s availability and changes dramatically after TPEN treatment (Higo et al., 2005; Watanabe et al., 2019). To rule out the possibility that TPEN suppresses the function of IP3R1 by modifying the subcellular distribution of ERp44, we overexpressed ERp44 by injecting mRNA encoding HA-tagged ERp44 into MII eggs and monitored the effect on Ca2+ release. TPEN did not alter the localization of ERp44 (Figure 3—figure supplement 1), and overexpression of ERp44 modified neither the Ca2+ oscillations induced by agonists (Figure 3—figure supplement 1) nor the effectiveness of TPEN to block them (data not shown). Thus, TPEN and Zn2+ deficiency most likely inhibits Ca2+ release by directly interfering with IP3R1 function rather than modifying this particular regulator.
Zn2+ depletion diminishes the ER Ca2+ leak and increases Ca2+ store content
Our above cIP3 results that TPEN inhibited IP3R1-mediated Ca2+ release and interrupted in-progress Ca2+ rises despite the presence of high levels of environmental IP3 suggest its actions are probably independent of IP3 binding, agreeing with an earlier report showing that TPEN did not modify IP3’s affinity for the IP3R (Richardson and Taylor, 1993). Additionally, the presence of a Zn2+-binding motif near the C-term cytoplasmic domain of the IP3R1’s channel, which is known to influence agonist-induced IP3R1 gating (Fan et al., 2015), led us to posit and examine that Zn2+ deficiency may be disturbing Ca2+ release to the cytosol and out of the ER. To probe this possibility, we queried if pretreatment with TPEN inhibited Ca2+ release through IP3R1. We first used thapsigargin (Tg), a sarcoplasmic/ER Ca2+ ATPase pump inhibitor (Thastrup et al., 1990) that unmasks a constitutive Ca2+ leak out of the ER (Lemos et al., 2021); in eggs, we have demonstrated it is mediated at least in part by IP3R1 (Wakai et al., 2019). Treatment with TPEN for 15 min slowed the Tg-induced Ca2+ leak into the cytosol, resulting in delayed and lowered amplitude Ca2+ responses (Figure 4A; p<0.05). To test whether the reduced response to Tg means that TPEN prevented the complete response of Tg, leaving a temporarily increased Ca2+ content in the ER, we added the Ca2+ ionophore ionomycin (Io), which empties all stores independently of IP3Rs. Io-induced Ca2+ responses were 3.3-fold greater in TPEN-treated cells, supporting the view that TPEN interferes with the ER Ca2+ leak (Figure 4A; p<0.05). We further evaluated this concept using in vitro aged eggs that often display reduced Ca2+ store content than freshly collected counterparts (Abbott et al., 1998). After culturing eggs in the presence or absence of TPEN for 2 hr, we added Io during Ca2+ monitoring, which in TPEN-treated eggs induced bigger Ca2+ rises than in control eggs (Figure 4B; p<0.05). We confirmed that this effect was independent of IP3R1 degradation because TPEN did not change IP3R1 reactivity in unfertilized eggs (Figure 4C; p<0.05).
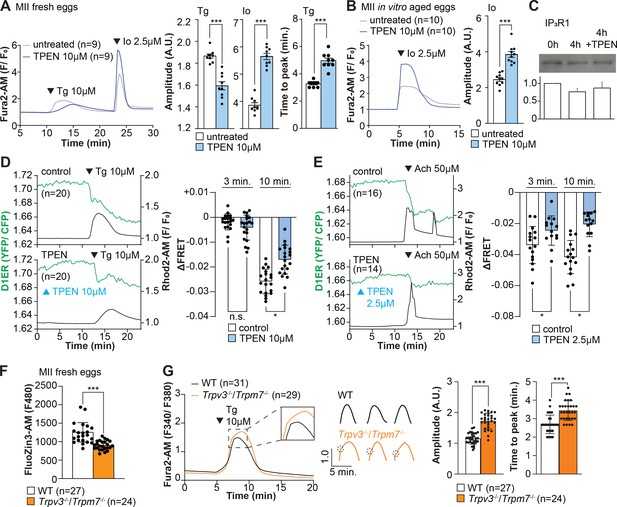
Zn2+ depletion alters Ca2+ homeostasis and increases Ca2+ store content independent of IP3R1 mass.
(A, B) Representative Ca2+ traces of MII eggs after the addition of Tg and Io in the presence or absence of TPEN. Blue trace recordings represent TPEN-treated eggs, whereas gray traces represent control, untreated eggs. (A) Io was added to fresh MII eggs once Ca2+ returned to baseline after treatment with Tg. Comparisons of mean peak amplitudes after Tg and Io are shown in the bar graphs in the right panel (p<0.001). (B) MII eggs were aged by 2 hr. incubation supplemented or not with TPEN followed by Io addition and Ca2+ monitoring (p<0.001). (C) Western blot showing the intensities of IP3R1 bands in MII eggs freshly collected, aged by 4 hr. incubation without TPEN, and with TPEN. Thirty eggs per lane in all cases. This experiment was repeated three times. (D, E) Left panels: representative traces of Ca2+ values in eggs loaded with the Ca2+-sensitive dye Rhod-2 AM and the ER Ca2+reporter, D1ER (1 µg/µl mRNA). TPEN was added into the media followed 10 min later by (D) 10 µM Tg and (E) 50 µM Ach. Right panel: bars represent the difference of FRET value between at the time of Tg/ Ach addition and at 3 and 5 min later of the addition (p<0.05). Experiments were repeated two different times for each treatment. Black and green traces represent cytosolic Ca2+ and Ca2+-ER, respectively. Blue and black arrowheads indicate the time of addition of TPEN and Tg/Ach, respectively. (F) Basal Zn2+ level comparison in WT (open bar) and Trpv3-/-/Trpm7-/- (dKO, orange bar) MII eggs. Each plot represents the Fluozin3 measurement at 5 min after starting monitoring. (G) Left panel: representative Ca2+ traces of WT (black trace) and dKO (orange trace) MII eggs after adding Tg. Insets represent the magnified traces at the peak of Ca2+ spike from different sets of eggs. Middle panel: individual traces of WT and dKO eggs after Tg addition. Dashed circles represent the flection point in dKO traces. Right panel: comparisons of mean peak amplitudes after Tg and the time between Tg addition and the Ca2+ peak are shown in the bar graphs in the right panel (p<0.001).
-
Figure 4—source data 1
IP3R1 and TPEN western blotting.
- https://cdn.elifesciences.org/articles/88082/elife-88082-fig4-data1-v1.zip
Next, we used the genetically encoded FRET sensor D1ER (Palmer et al., 2004) to assess the TPEN’s effect on the ER’s relative Ca2+ levels changes following the additions of Tg or Ach. TPEN was added 10 min before 10 µM Tg or 50 µM Ach, and we simultaneously monitored changes in cytosolic and intra-ER Ca2+ (Figure 4D and E). For the first 3 min, the Tg-induced decrease in Ca2+-ER was similar between groups. However, while the drop in Ca2+ content continued in control eggs, in TPEN-treated eggs, it came to an abrupt halt, generating profound differences between the two groups (Figure 4D; p<0.05). TPEN had even more pronounced effects following the addition of Ach, leading to a reduced- and prematurely terminated Ca2+ release from the ER in treated eggs (Figure 4E; p<0.05).
Lastly, we sought to use a cellular model where low labile Zn2+ occurred without pharmacology. To this end, we examined a genetic model where the two non-selective plasma membrane channels that could influx Zn2+ in maturing oocytes have been deleted (Bernhardt et al., 2017; Carvacho et al., 2016; Carvacho et al., 2013), namely, the transient receptor potential melastatin-7 (TRPM7) and TRP vanilloid 3 (TRPV3), both members of the TRP superfamily of channels (Wu et al., 2010). We found that eggs from double knockout females (dKOs) had lower levels of labile Zn2+ (Figure 4F), and the addition of Tg revealed an expanded Ca2+ store content in these eggs vs. control WT eggs (Figure 4G). Remarkably, in dKO eggs, the Ca2+ rise induced by Tg showed a shoulder or inflection point before the peak delaying the time to peak (Figure 4G, inset; p<0.001). These results in dKO eggs show a changed dynamic of the Tg-induced Ca2+ release, suggesting that lower levels of labile Zn2+ modify ER Ca2+ release independently of chelators.
Ca2+ oscillations in eggs occur within a window of Zn2+ concentrations
We next examined whether resupplying Zn2+ could restart the Ca2+ oscillations terminated by Zn2+ depletion. Zn pyrithione (ZnPT) rapidly increases cellular Zn2+ upon extracellular addition (Barnett et al., 1977; Robinson, 1964). Dose titration studies and imaging fluorimetry revealed that 0.01 µM ZnPT caused subtle and protracted increases in Zn2+ levels, whereas 0.1 µM ZnPT caused rapid increases in eggs’ Zn2+ baseline (Figure 5A). We induced detectable Ca2+ oscillations by injection of mPlcz1 mRNA followed by 50 µM TPEN (Figure 5B), which terminated them. After 30 min, we added 0.1 µM ZnPT, and within 15 min the oscillations restarted in most TPEN-treated eggs (Figure 5C). We repeated this approach using thimerosal (Figure 5D and E). Adding 0.1 µM ZnPT did not restore the Ca2+ oscillations retrained by TPEN, but 0.5 µM ZnPT did so (Figure 5E). These results demonstrate that Zn2+ plays a pivotal, enabling role in the generation of Ca2+ oscillations in mouse eggs.
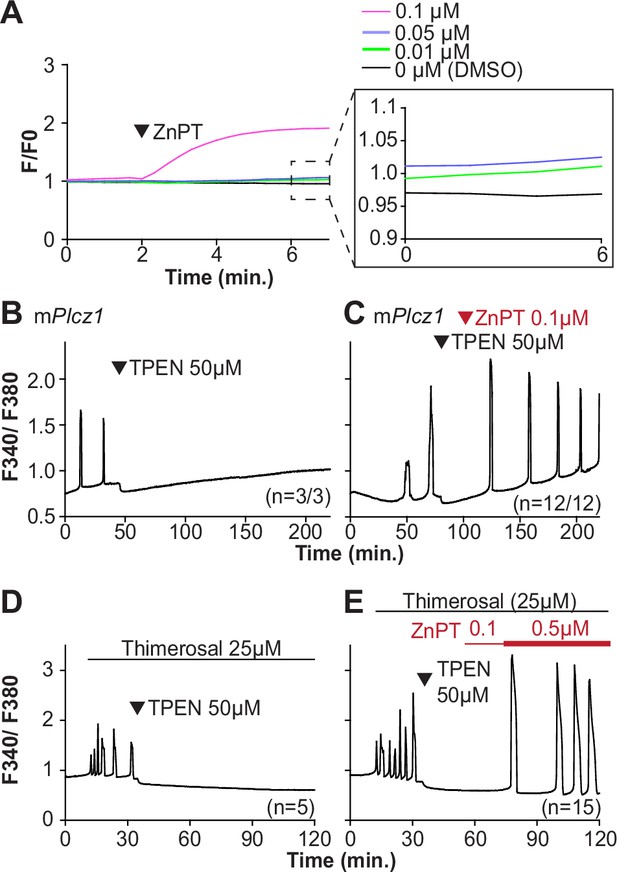
Restoring Zn2+ levels with ZnPT rescues oscillations interrupted by TPEN-induced Zn2+ deficiency.
(A) Representative traces of Zn2+ in MII eggs following the addition of 0.01–0.1 µM concentrations of ZnPT. The broken rectangular area is amplified in the next panel to appreciate the subtle increase in basal Zn2+ caused by the addition of ZnPT. (B, C) mPlcz1 mRNA (0.01 µg/µl)-induced oscillations followed by the addition of TPEN (black arrowhead) (B), or after the addition of TPEN followed by ZnPT (red arrowhead) (C). (D, E) Thimerosal (25 µM) induced oscillations using the same sequence of TPEN (D) and ZnPT (E), but higher concentrations of ZnPT were required to rescue thimerosal-initiated oscillations (E). These experiments were repeated at least two different times.
Excessive intracellular Zn2+ inhibits Ca2+ oscillations
Zn2+ is necessary for diverse cellular functions, consistent with numerous amino acids and proteins capable of binding Zn2+ within specific and physiological ranges (Pace and Weerapana, 2014). Excessive Zn2+, however, can cause detrimental effects on cells and organisms (Broun et al., 1990; Hara et al., 2022; Sikora and Ouagazzal, 2021). Consistent with the deleterious effects of Zn2+, a previous study showed that high concentrations of ZnPT, ~50 µM, prevented SrCl2-induced egg activation and initiation of development (Bernhardt et al., 2012; Kim et al., 2011). We examined how ZnPT and excessive Zn2+ levels influence Ca2+ oscillations. Our conditions revealed that pre-incubation or continuous exposure to 0.1 µM or 1.0 µM ZnPT delayed or prevented egg activation induced by mPlcz1 mRNA injection (Figure 6—figure supplement 1). We used these ZnPT concentrations to add it into ongoing oscillations induced by ICSI and monitored the succeeding Ca2+ responses. The addition of 0.05–10 µM ZnPT caused an immediate elevation of the basal levels of Fura-2 and termination of the Ca2+ oscillations (Figure 6A–D). mPlcz1 mRNA-initiated Ca2+ responses were also interrupted by adding 0.1 µM ZnPT, whereas untreated eggs continued oscillating (Figure 6E and F). ZnPT also inhibited IP3R1-mediated Ca2+ release triggered by cIP3, suggesting that excessive Zn2+ directly inhibits IP3R1 function (Figure 6G).
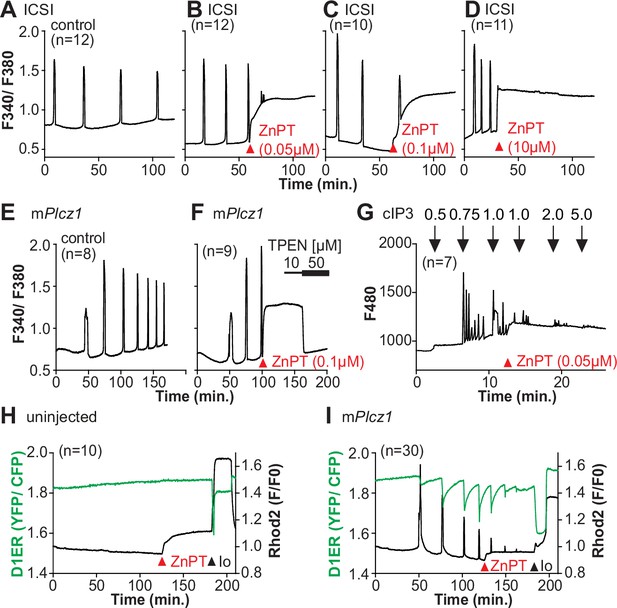
Excess Zn2+ hinders Ca2+ oscillations.
(A–D) Intracytoplasmic sperm injection (ICSI)-initiated Ca2+ response without (A) or following the addition of ZnPT (B, C) (the time of ZnPT addition and concentration are denoted above the tracing). (E, F) Representative Ca2+ responses induced by injection of 0.01 µg/µl mPlcz1 mRNA in untreated eggs (E) or in eggs treated with 0.1 µM ZnPT followed by 10 µM TPEN first and then 50 µM (F). (G) cIP3-induced Ca2+ release as expected when the UV pulses in the absence but not in the presence of 0.05 µM ZnPT (the time of addition is denoted by a bar above the tracing). (H, I) Representative traces of Ca2+ values in eggs loaded with the Ca2+-sensitive dye Rhod-2 AM and the ER Ca2+reporter, D1ER (1 µg/µl mRNA). Uninjected and 0.01 µg/µl mPlcz1 mRNA-injected eggs were monitored. After initiation and establishment of the oscillations, 0.1 µM ZnPT was added into the media followed 30 min later by 2.5 µM Io. Experiments were repeated two different times. Red and black arrowheads indicate the time of addition of ZnPT and Io, respectively.
A noticeable feature of ZnPT is the increased basal ratios of Fura-2 AM. These changes could reflect enhanced IP3R1 function and increased basal Ca2+ concentrations caused by Zn2+ stimulation of IP3R1. This seems unlikely, however, because extended elevated cytosolic Ca2+ would probably induce cellular responses, such as the release of the second polar body, egg fragmentation, or cell death, neither of which happened. It might reflect, instead, Fura-2’s ability to report changes in Zn2+ levels, which seemed the case because the addition of TPEN lowered fluorescence without restarting the Ca2+ oscillations (Figure 6F). To ensure the impact of ZnPT abolishing Ca2+ oscillations was not an imaging artifact obscuring ongoing rises, we simultaneously monitored cytoplasmic and ER Ca2+ levels with Rhod-2 and D1ER, respectively. This approach allowed synchronously observing Ca2+ changes in both compartments that should unfold in opposite directions. In control, uninjected eggs, the fluorescent values for both reporters remained unchanged during the monitoring period, whereas in mPlcz1 mRNA-injected eggs, the reporters’ signals displayed simultaneous but opposite changes, as expected (Figure 6H and I). The addition of ZnPT in uninjected eggs rapidly increased Rhod-2 signals but not D1ER’s, which was also the case in oscillating eggs, as the addition of ZnPT did not immediately alter the dynamics of the ER’s Ca2+ release, suggesting that D1ER faithfully reports in Ca2+ changes but cannot detect changes in Zn2+ levels, at least to this extent; ZnPT progressively caused fewer and lower amplitude changes in D1ER fluorescence, consistent with the diminishing and eventual termination of the Ca2+ oscillations. Noteworthy, in these eggs, the basal D1ER fluorescent ratio remained unchanged after ZnPT, demonstrating its unresponsiveness to Zn2+ changes of this magnitude. The ZnPT-induced increases in Rhod-2 fluorescence without concomitant changes in D1ER values suggest that the changes in the dyes’ fluorescence do not represent an increase in basal Ca2+ and, more likely, signal an increase in intracellular Zn2+. We confirmed that both reporters were still in working order as the addition of Io triggered Ca2+ changes detected by both reporters (Figure 6H and I).
Discussion
This study demonstrates that appropriate levels of labile Zn2+ are essential for initiating and maintaining IP3R1-mediated Ca2+ oscillations in mouse eggs regardless of the initiating stimuli. Both deficient and excessive Zn2+ compromise IP3R1 sensitivity, diminishing and mostly terminating Ca2+ oscillations. The results demonstrate that IP3R1 and Zn2+ act in concert to modulate Ca2+ signals, revealing previously unexplored crosstalk between these ions at fertilization (Figure 7).
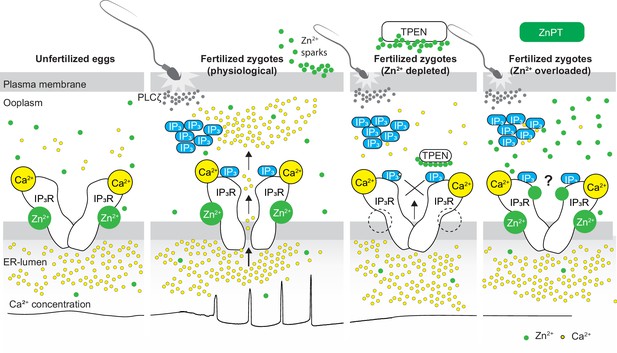
Schematic of proposed regulation of IP3R1 function by Zn2+ in eggs and fertilized zygotes.
In MII eggs, left panel, IP3R1s are in a Ca2+-release permissive state with optimal levels of cytoplasmic Ca2+ and Zn2+ and maximum endoplasmic reticulum (ER) content, but Ca2+ is maintained at resting levels by the combined actions of pumps, ER Ca2+ leak, and reduced influx. Once fertilization takes place, left center panel, robust IP3 production induced by the sperm-borne PLCζ leads to Ca2+ release through ligand-induced gating of IP3R1. Continuous IP3 production and refilling of the stores via Ca2+ influx ensure the persistence of the oscillations. Zn2+ release occurs in association with first few Ca2+ rises and cortical granule exocytosis, Zn2+ sparks, lowering Zn2+ levels but not sufficiently to inhibit IP3R1 function. Zn2+ deficiency caused by TPEN or other permeable Zn2+ chelators, right center panel, dose-dependently impairs IP3R1 function and limits Ca2+ release. We propose this is accomplished by stripping the Zn2+ bound to the residues of the zinc-finger motif in the LNK domain of IP3R1 that prevents the allosteric modulation of the gating process induced by IP3 or other agonists. We propose that excess Zn2+, right panel, also inhibits IP3R1-mediate Ca2+ release, possibly by non-specific binding of thiol groups present in cysteine residues throughout the receptor (denoted by a?). We submit that optimal Ca2+ oscillations in mouse eggs unfold in the presence of a permissive range of Zn2+ concentration.
Zn2+ is an essential micronutrient for living organisms (Kaur et al., 2014) and is required for various cellular functions, such as proliferation, transcription, and metabolism (Lo et al., 2020; Maret and Li, 2009; Yamasaki et al., 2007). Studies using Zn2+ chelators have uncovered what appears to be a cell-specific, narrow window of Zn2+ concentrations needed for cellular proliferation and survival (Carraway and Dobner, 2012; Lo et al., 2020). Further, TPEN appeared especially harmful, and in a few cell lines, even low doses provoked oxidative stress, DNA fragmentation, and apoptosis (Mendivil-Perez et al., 2012). We show here that none of the Zn2+ chelators, permeable or impermeable, affected cell viability within our experimental observations, confirming findings from previous studies that employed high concentrations of TPEN to interrupt the Ca2+ oscillations (Lawrence et al., 1998) or inducing egg activation of mouse eggs (Suzuki et al., 2010b). Our data demonstrating that ~2.5 µM is the threshold concentration of TPEN in eggs that first causes noticeable changes in basal Zn2+, as revealed by FluoZin, is consistent with the ~2–5 µM Zn2+ concentrations in most culture media without serum supplementation (Lo et al., 2020), and with the ~100 pM basal Zn2+ in cells (Qin et al., 2011). Lastly, the effects on Ca2+ release observed here with TPEN and other chelators were due to the chelation of Zn2+, as pretreatment with ZnSO4 but not with equal or greater concentrations of MgCl2 or CaCl2 rescued the inhibition of the responses, which is consistent with results by others (Kim et al., 2010; Lawrence et al., 1998).
To identify how Zn2+ deficiency inhibits Ca2+ release in eggs, we induced Ca2+ oscillations using various stimuli and tested the effectiveness of membrane-permeable and -impermeable chelators to abrogate them. Chelation of extracellular Zn2+ failed to terminate the Ca2+ responses, whereas membrane-permeable chelators did, pointing to intracellular labile Zn2+ levels as essential for Ca2+ release. All agonists used here were susceptible to inhibition by TPEN, whether their activities depended on IP3 production or allosterically induced receptor function, although the effective TPEN concentrations varied across stimuli. Some agents, such as mPlcz1 mRNA or thimerosal, required higher concentrations than SrCl2, Ach, or cIP3. The reason underlying the different agonists’ sensitivities to TPEN will require additional research, but the persistence of IP3 production or change in IP3R1 structure needed to induce channel gating might explain it. However, the universal abrogation of Ca2+ oscillations by TPEN supports the view drawn from cryo-EM-derived IP3R1 models that signaling molecules can allosterically induce channel gating from different starting positions in the receptor by mechanically coupling the binding effect to the ion-conducting pore in the C-terminal end of IP3R (Fan et al., 2015). The cytosolic C-terminal domain of each IP3R1 subunit is alongside the IP3-binding domain of another subunit and, therefore, well positioned to sense IP3 binding and induce channel gating (Fan et al., 2015). Within each subunit, the LNK domain, which contains a Zn2+-finger motif (Fan et al., 2015), connects the opposite domains of the molecule. Although there are no reports regarding the regulation of IP3R1 sensitivity by Zn2+, such evidence exists for RyRs (Woodier et al., 2015), which also display a conserved Zn2+-finger motif (des Georges et al., 2016). Lastly, mutations of the two Cys or two His residues of this motif, without exception, resulted in inhibition or inactivation of the IP3R1 channel (Bhanumathy et al., 2012; Uchida et al., 2003). These results are consistent with the view that the C-terminal end of IP3Rs plays a dominant role in channel gating (Bhanumathy et al., 2012; Uchida et al., 2003). We propose that TPEN inhibits Ca2+ oscillations in mouse eggs because chelating Zn2+ interferes with the function of the LNK domain and its Zn2+-finger motif proposed role on the mechanical coupling induced by agonist binding to the receptor that propagates to the pore-forming region and required to gate the channel’s ion-pore (Fan et al., 2022; Fan et al., 2015).
In support of this possibility, TPEN-induced Zn2+-deficient conditions altered the Ca2+-releasing kinetics in resting eggs or after fertilization. Tg increases intracellular Ca2+ by inhibiting the SERCA pump (Thastrup et al., 1990) and preventing the reuptake into the ER of the ebbing Ca2+ during the basal leak. Our previous studies showed that the downregulation of IP3R1 diminishes the leak, suggesting that it occurs through IP3R1 (Wakai and Fissore, 2019). Consistent with this view, TPEN pretreatment delayed the Ca2+ response induced by Tg, implying that Zn2+ deficiency hinders Ca2+ release through IP3R1. An expected consequence would be increased Ca2+ content in the ER after Tg. Io that mobilizes Ca2+ independently of IP3Rs (Toeplitz et al., 1979) induced enhanced responses in TPEN-treated eggs vs. controls, confirming the accumulation of Ca2+- ER in Zn2+-deficient conditions. We demonstrated that this accumulation is due to hindered emptying of the Ca2+ ER evoked by agonists in Zn2+-deficient environments, resulting in reduced cytosolic Ca2+ increases, as IP3R1 is the pivotal intermediary channel between these compartments. Noteworthy, the initial phase of the Tg-induced Ca2+ release out of the ER did not appear modified by TPEN, as if it was mediated by a Zn2+-insensitive Ca2+ channel(s)/transporter, contrasting with the abrogation of Ach-induced ER emptying from the outset. Remarkably, independently of Zn2+ chelators, emptying of Ca2+ ER was modified in a genetic model of Zn2+-deficient oocytes lacking two TRP channels, confirming the impact of Zn2+ on Ca2+ release. It is worth noting that TPEN did not reduce but maintained or increased the mass of IP3R1, which might result in the inhibition of Zn2+-dependent ubiquitin ligase Ubc7 by the Zn-deficient conditions (Webster et al., 2003). We cannot rule out that these conditions may undermine other conformational changes required to trigger IP3R1 degradation, thereby favoring the accumulation of IP3R1.
Despite accruing Zn2+ during oocyte maturation, fertilization witnesses a necessary Zn2+ release into the external milieu, known as ‘Zn2+ sparks’ (Converse and Thomas, 2020; Kim et al., 2011; Mendoza et al., 2022; Que et al., 2019; Que et al., 2015; Seeler et al., 2021). This release of Zn2+ is a conserved event in fertilization across species and is associated with several biological functions, including those related to fending off polyspermy (Kim et al., 2011; Que et al., 2019; Wozniak et al., 2020). The concomitant decrease in Zn2+ facilitates the resumption of the cell cycle and exit from the MII stage (Kim et al., 2011). Congruent with this observation, artificial manipulation that maintains high Zn2+ levels prevents egg activation (Kim et al., 2011), whereas lowering Zn2+ with chelators leads to egg activation without Ca2+ mobilization (Suzuki et al., 2010b). As posed by others, these results suggest that meiosis completion and the early stages of fertilization unfold within a narrow window of permissible Zn2+ (Kim et al., 2011; Kim et al., 2010). Here, we extend this concept and show that IP3R1 function and the Ca2+ oscillations in mouse eggs require this optimal level of labile Zn2+ because the Ca2+ responses interrupted by TPEN-induced Zn2+-insufficiency are rescued by restoring Zn2+ levels with ZnPT. Furthermore, unopposed increases in Zn2+ by exposure to ZnPT abrogated fertilization-initiated Ca2+ oscillations and prevented the expected egg activation events. It is unclear how excess Zn2+ disturbs the function of IP3R1. Nevertheless, IP3R1s have multiple cysteines whose oxidation enhances the receptor sensitivity to IP3 (Joseph et al., 2018), and it is possible that excessive Zn2+ aberrantly modifies them, disturbing IP3R1 structure and function or, alternatively, preventing their oxidation and sensitization of the receptor. Lastly, we cannot rule out that high Zn2+ levels directly inhibit the receptor’s channel. These results reveal a close association between the Zn2+ levels controlling meiotic transitions and the Ca2+ release necessary for egg activation, placing the IP3R1 at the center of the crosstalk of these two divalent cations.
Abrupt Zn2+ changes have emerged as critical signals for meiotic and mitotic transitions in oocytes, eggs, embryos, and somatic cells (Kim et al., 2011; Kim et al., 2010; Lo et al., 2020). Fertilization relies on prototypical Ca2+ rises and oscillations, and Zn2+ sparks are an egg activation event downstream of this Ca2+ release, establishing a functional association between these two divalent cations that continues to grow (Kim et al., 2011). Here, we show that, in addition, these cations actively crosstalk during fertilization and that the fertilization-induced Ca2+ oscillations rely on optimized IP3R1 function underpinned by ideal Zn2+ levels set during oocyte maturation. Future studies should explore if artificial alteration of Zn2+ levels can extend the fertile lifespan of eggs, improve developmental competence, or develop methods of non-hormonal contraception.
Materials and methods
Reagent type (species) or resource | Designation | Source or reference | Identifiers | Additional information |
---|---|---|---|---|
Genetic reagent (Mus musculus) | CD1 | Charles River | 022 | |
Genetic reagent (M. musculus) | C57BL/6J | JAX | JAX: 000664 | |
Genetic reagent (M. musculus) | Trpm7-floxed | A generous gift from Dr. Carmen P. Williams (NIEHS) (PMID:30322909) | C57BL6/J and 129s4/SvJae mixed background | |
Genetic reagent (M. musculus) | Gdf9-cre | JAX | JAX: 011062 | |
Genetic reagent (M. musculus) | Trpv3−/− | A generous gift from Dr H. Xu (PMID:20403327) | C57BL/6J and 129/SvEv mixed background | |
Biological sample (mouse oocyte) | Mus musculus | This paper | Eggs at the metaphase of the second meiosis | |
Biological sample (mouse sperm) | Mus musculus | This paper | Matured sperm from cauda epididymis | |
Recombinant DNA reagent | pcDNA6-mouse Plcz1-venus (plasmid used as a template for mRNA synthesis) | Published in previous Fissore lab paper PMID: 34313315 Mouse Plcz1 sequence was a generous gift from Dr. Kiyoko Fukami (PMID:18028898) | Mouse Plcz1 mRNA was fused with Venus and inserted in pcDNA6 vector | |
Recombinant DNA reagent | pcDNA6-CALR-D1ER-KDEL (plasmid used as a template for mRNA synthesis) | Published in previous Fissore lab paper PMID:24101727 Original D1ER vector was a generous gift from Dr. Roger Y Tsien (PMID:15585581) | FRET construct D1ER was inserted between ER-targeting sequence of calreticulin and KDEL ER retention signal in pcDNA6 vector | |
Recombinant DNA reagent | pcDNA6-human ERP44-HA (plasmid used as a template for mRNA synthesis) | This paper Original human ERp44 sequence was a generous gift from Dr. Roberto Sitia (PMID:11847130) | Human ERP44 mRNA fused with HA in pcDNA6/Myc His B vector | |
Antibody | Monoclonal HA (mouse monoclonal) | Roche | 11581816001 | Dilution: 1:200 |
Antibody | Polyclonal IP3R1 (rabbit polyclonal) | Parys et al., 1995 | Dilution: 1:1000 | |
Antibody | Monoclonal α-tubulin (mouse monoclonal) | Sigma-Aldrich | T-9026 | Dilution: 1:1000 |
Antibody | Alexa Fluor 488 (goat polyclonal) | Invitrogen | Invitrogen: A32723 | Dilution: 1:400 |
Commercial assay or kit | T7 mMESSAGE mMACHINE Kit | Invitrogen | Invitrogen: AM1344 | Used for in vitro mRNA synthesis |
Commercial assay or kit | Poly(A) Tailing Kit | Invitrogen | Invitrogen: AM1350 | Used for poly (A) tailing of synthesized mRNA |
Chemical compound, drug | Hyaluronidase from bovine testes | Sigma-Aldrich | H3506 | |
Chemical compound, drug | 3-Isobutyl-1-methylxanthine (IBMX) | Sigma-Aldrich | I5879 | |
Chemical compound, drug | Polyvinylpyrrolidone (PVP) (average molecular weight: 360,000) | Sigma-Aldrich | PVP360 | Used for mRNA microinjection and ICSI |
Chemical compound, drug | N,N, N′,N′-Tetrakis (2-pyridylmethyl) ethylenediamine (TPEN) | Sigma-Aldrich | P4413 | Prepared in DMSO and kept at –20°C until use |
Chemical compound, drug | Zinc pyrithione (ZnPT) | Sigma-Aldrich | PHR1401 | Prepared in DMSO and kept at –20°C until use |
Chemical compound, drug | Strontium chloride hexahydrate (SrCl2) | Sigma-Aldrich | 255521 | Freshly dissolved in water on the day of experiment |
Chemical compound, drug | Calcium chloride dihydrate (CaCl2) | Sigma-Aldrich | C3881 | Freshly dissolved in water on the day of experiment |
Chemical compound, drug | Magnesium chloride hexahydrate (MgCl2) | Sigma-Aldrich | M2393 | Freshly dissolved in water on the day of experiment |
Chemical compound, drug | Zinc sulfate monohydrate (ZnSO4) | Acros Organics | 389802500 | Freshly dissolved in water on the day of experiment |
Chemical compound, drug | Ethylenediaminetetraacetic acid sodium dihydrate (EDTA) | LabChem | LC137501 | Prepared as 0.5 M aqueous solution with pH 8.0 adjusted by NaOH |
Chemical compound, drug | Diethylenetriaminepentaacetic acid (DTPA) | Sigma-Aldrich | D6518 | |
Chemical compound, drug | Tris (2-pyridylmethyl) amine (TPA) | Santa Cruz | sc-477037 | |
Chemical compound, drug | Dimethyl sulfoxide (DMSO) | Sigma-Aldrich | D8418 | Used as a solvent |
Chemical compound, drug | Acetylcholine chloride | Sigma-Aldrich | A6625 | |
Chemical compound, drug | Thimerosal | Sigma-Aldrich | T5125 | Freshly dissolved in water on the day of experiment and kept on ice until use |
Chemical compound, drug | Ionomycin calcium salt | Tocris | 1704 | Working concentration: 2.5 µM |
Chemical compound, drug | Thapsigargin | Calbiochem | #586500 | Working concentration: 10 µM |
Other | Pluronic F-127 (20% solution in DMSO) (pluronic acid) | Invitrogen | P3000MP | Added to dye dilutions to facilitate the solubilization |
Other | Fura-2 AM | Invitrogen | F1221 | Ratiometric fluorescent Ca2+ indicator Used at 1.25 µM in TL-HEPES containing 0.02% pluronic acid |
Other | FluoZin-3 AM | Invitrogen | F24195 | Fluorescent Zn2+ indicator Used at 1.25 µM in TL-HEPES containing 0.02% pluronic acid |
Other | Fluo-4 AM | Invitrogen | F14201 | Fluorescent Ca2+ indicator Used at 1.25 µM in TL-HEPES containing 0.02% pluronic acid |
Other | Rhod2-AM | Invitrogen | R1244 | Fluorescent Ca2+ indicator Used at 2.2 µM in TL-HEPES containing 0.02% pluronic acid. |
Other | ci-IP3/PM | Tocris | 6210 | Photo-activatable IP3. Dissolved in DMSO and kept at –20°C Before use, the stock was diluted with water to make a final concentration of 0.25 mM |
Other | Pme1 | New England BioLabs | R0560S | Used to linearize pcDNA6 vectors for mRNA synthesis |
Software, algorithm | Prism | GraphPad Software | Version 5.01 |
N,N,N′,N′-tetrakis (2-pyridinylmethyl)–1,2-ethylenediamine (TPEN) and zinc pyrithione (ZnPT) were dissolved in dimethyl sulfoxide (DMSO) at 10 mM and stored at –20°C until use. SrCl2, CaCl2, ZnSO4, and MgCl2 were freshly dissolved with double-sterile water at 1 M and diluted with the monitoring media just before use. Ethylenediaminetetraacetic acid (EDTA) and diethylenetriaminepentaacetic acid (DTPA) were reconstituted with double-sterile water at 0.5 M and 10 mM, respectively, and the pH was adjusted to 8.0. Tris(2-pyridylmethyl) amine (TPA) was diluted in DMSO at 100 mM and stored at –20°C until use. Acetylcholine chloride and thimerosal were dissolved in double-sterile water at 550 mM and 100 mM, respectively. Acetylcholine was stored at –20°C until use, whereas thimerosal was made fresh in each experiment.
Mice
The University of Massachusetts Institutional Animal Care and Use Committee (IACUC) approved all animal experiments and protocols. Trpm7-floxed (Trpm7fl/fl) Gdf9-Cre and Trpv3−/− mice were bred at our facility. Trpm7fl/fl mice were crossed with Trpv3−/− to generate Trpm7fl/fl; Trpv3−/− mouse line. Female Trpm7fl/fl; Trpv3−/− mice were crossed with Trpm7fl/fl; Trpv3−/−; Gdf9-cre male to generate females null for Trpv3 and with oocyte-specific deletion for Trpm7. Ear clips from offspring were collected prior to weaning, and confirmation of genotype was performed after most experiments.
Egg collection
Request a detailed protocolAll gamete handling procedures are as previously reported by us (Wakai et al., 2019). MII eggs were collected from the ampulla of 6- to 8-week-old female mice. Females were superovulated via intraperitoneal injections of 5 IU pregnant mare serum gonadotropin (PMSG, Sigma, St. Louis, MO) and 5 IU human chorionic gonadotropin (hCG, sigma) at 48 hr interval. Cumulus-oocyte-complexes (COCs) were obtained 13.5 hr post-hCG injection by tearing the ampulla using forceps and needles in TL-HEPES medium. COCs were treated with 0.26% (w/v) of hyaluronidase at room temperature (RT) for 5 min to remove cumulus cells.
Intracytoplasmic sperm injection (ICSI)
Request a detailed protocolICSI was performed as previously reported by us (Kurokawa and Fissore, 2003) using described setup and micromanipulators (Narishige, Japan). Sperm from C57BL/6 or CD1 male mice (7–12 weeks old) were collected from the cauda epididymis in TL-HEPES medium, washed several times, heads separated from tails by sonication (XL2020; Heat Systems Inc, USA) for 5 s at 4°C. The sperm lysate was washed in TL-HEPES and diluted with 12% polyvinylpyrrolidone (PVP, MW = 360 kDa) to a final PVP concentration of 6%. A piezo micropipette-driving unit was used to deliver the sperm into the ooplasm (Primetech, Ibaraki, Japan); a few piezo-pulses were applied to puncture the eggs’ plasma membrane following penetration of the zona pellucida. After ICSI, eggs were either used for Ca2+ monitoring or cultured in KSOM to evaluate activation and development at 36.5°C in a humidified atmosphere containing 5% CO2.
Preparation and microinjection of mRNA
Request a detailed protocolpcDNA6-mPlcz1-mEGFP, pcDNA6-CALR-D1ER-KDEL, and pcDNA6-humanERp44-HA were linearized with the restriction enzyme PmeI and in vitro transcribed using the T7 mMESSAGE mMACHINE Kit following procedures previously used in our laboratory (Ardestani et al., 2020). A poly(A) tail was added to the in vitro synthesized RNA (mRNA) using Tailing Kit followed by quantification and dilution to 0.5 μg/μl in nuclease-free water and stored at –80°C until use. Before microinjection, mPlcz1, D1ER, and ERp44 mRNA were diluted to 0.01, 1.0, and 0.5 μg/μl, respectively, in nuclease-free water, heated at 95°C for 3 min followed by centrifugation at 13,400 × g for 10 min at 4°C. Cytoplasm injection of mRNA was performed under microscopy equipped with micromanipulators (Narishige, Japan). The zona pellucida and the plasma membrane of MII eggs were penetrated by applying small pulses generated by the piezo micromanipulator (Primetech). The preparation of the injection pipette was as for ICSI (Kurokawa and Fissore, 2003), but the diameter of the tip was ~1 μm.
Ca2+ and Zn2+ imaging
Request a detailed protocolBefore Ca2+ imaging, eggs were incubated in TL-HEPES containing 1.25 μM Fura2-AM, 1.25 μM FluoZin3-AM, or 2.2 μM Rhod2-AM and 0.02% pluronic acid for 20 min at RT and then washed. The fluorescent probe-loaded eggs were allowed to attach to the bottom of the glass dish (Mat-Tek Corp., Ashland, MA). Eggs were monitored simultaneously using an inverted microscope (Nikon, Melville, NY) outfitted for fluorescence measurements. Fura-2 AM, FluoZin3-AM, and Rhod2-AM fluorescence were excited with 340 nm and 380 nm, 480 nm, and 550 nm wavelengths, respectively, every 20 s, for different intervals according to the experimental design and as previously performed in the laboratory. The illumination was provided by a 75 W Xenon arc lamp and controlled by a filter wheel (Ludl Electronic Products Ltd, Hawthorne, NY). The emitted light above 510 nm was collected by a cooled Photometrics SenSys CCD camera (Roper Scientific, Tucson, AZ). Nikon Element software coordinated the filter wheel and data acquisition. The acquired data were saved and analyzed using Microsoft Excel and GraphPad using Prism software (Ardestani et al., 2020). For Figures 1A, 4A–C—6H–I, values obtained from FluoZin3-AM, Fura2-AM, or Rhod2-AM recordings were divided by the average of the first five recordings for each treatment that was used as the F0.
To estimate relative changes in Ca2+-ER, emission ratio imaging of the D1ER (YFP/CFP) was performed using a CFP excitation filter, dichroic beamsplitter, CFP and YFP emission filters (Chroma Technology, Rockingham, VT; ET436/20X, 89007bs, ET480/40m, and ET535/30m). To measure Ca2+-ER and cytosolic Ca2+ simultaneously, eggs that had been injected with D1ER were loaded with Rhod-2AM, and CFP, YFP, and Rhod-2 intensities were collected every 20 s.
Caged IP3
Request a detailed protocolCaged-IP3/PM (cIP3) was reconstituted in DMSO and stored at –20°C until use. Before injection, cIP3 stock was diluted to 0.25 mM with water and microinjected as above. After incubation in KSOM media at 37°C for 1 hr, the injected eggs were loaded with the fluorophore, 1.25 μM Fluo4-AM, and 0.02% pluronic acid and handled as above for Fura-2 AM. The release of cIP3 was accomplished by photolysis using 0.5–5 s pulses at 360 nm wavelengths. Ca2+ imaging was as above, but Fluo4 was excited at 488 nm wavelength and emitted light above 510 nm collected as above.
Western blot analysis
Request a detailed protocolCell lysates from 20 to 50 mouse eggs were prepared by adding 2× Laemmli sample buffer. Proteins were separated on 5% SDS–PAGE gels and transferred to PVDF membranes (Millipore, Bedford, MA). After blocking with 5% fat-free milk + TBS, membranes were probed with the rabbit polyclonal antibody specific to IP3R1 (1:1000; a generous gift from Dr. Jan Parys, Katholieke Universiteit, Leuven, Belgium; Parys et al., 1995). Goat anti-rabbit antibody conjugated to horseradish peroxidase (HRP) was used as a secondary antibody (1:5000; goat anti-rabbit IgG [H+L] Cross-Adsorbed Secondary Antibody, HRP; Invitrogen, Waltham, MA). For detection of chemiluminescence, membranes were developed using ECL Prime (Sigma) and exposed for 1–3 min to maximum sensitivity film (VWR, Radnor, PA). Broad-range pre-stained SDS–PAGE molecular weight markers (Bio-Rad, Hercules, CA) were run in parallel to estimate the molecular weight of the immunoreactive bands. The same membranes were stripped at 50°C for 30 min (62.5 mM Tris, 2% SDS, and 100 mM 2-beta mercaptoethanol) and re-probed with anti-α-tubulin monoclonal antibody (1:1000).
Immunostaining and confocal microscopy
Request a detailed protocolImmunostaining was performed according to our previous study (Akizawa et al., 2021). After incubation with or without TPEN, MII eggs were fixed with 4% (w/v) paraformaldehyde in house-made phosphate-buffered saline (PBS) for 20 min at RT and then permeabilized for 60 min with 0.2% (v/v) Triton X-100 in PBS. Next, the eggs were blocked for 45 min with a blocking buffer containing 0.2% (w/v) skim milk, 2% (v/v) fetal bovine serum, 1% (w/v) bovine serum albumin, 0.1% (v/v) TritonX-100, and 0.75% (w/v) glycine in PBS. Eggs were incubated overnight at 4°C with mouse anti-HA antibody (1:200) diluted in blocking buffer. Eggs were washed in blocking buffer 3× for 10 min, followed by incubation at RT for 30 min with a secondary antibody, Alexa Fluor 488 goat anti-mouse IgG (H+L) (1:400) diluted in blocking buffer. Fluorescence signals were visualized using a laser-scanning confocal microscope (Nikon A1 Resonant Confocal with six-color TIRF) fitted with a 63×, 1.4 NA oil-immersion objective lens.
Statistical analysis
Request a detailed protocolComparisons for statistical significance of experimental values between treatments and experiments were performed in three or more experiments performed on different batches of eggs in most studies. Given the number of eggs needed, WB studies were repeated twice. Prism-GraphPad software was used to perform the statistical comparisons that include unpaired Student’s t-tests, Fisher’s exact test, and one-way ANOVA followed by Tukey’s multiple comparisons, as applicable, and the production of graphs to display the data. All data are presented as mean ± SD. Differences were considered significant at p<0.05.
Data availability
All data generated or analyzed during this study are included in the manuscript and supporting files. Source data files have been provided for Figures 2 and 4.
References
-
Structural characterization of bis(N-oxopyridine-2-thionato)zinc(II)Inorganic Chemistry 16:1834–1838.https://doi.org/10.1021/ic50174a002
-
The inositol trisphosphate/calcium signaling pathway in health and diseasePhysiological Reviews 96:1261–1296.https://doi.org/10.1152/physrev.00006.2016
-
Identification of functionally critical residues in the channel domain of inositol trisphosphate receptorsThe Journal of Biological Chemistry 287:43674–43684.https://doi.org/10.1074/jbc.M112.415786
-
The thiol reagent, thimerosal, evokes Ca2+ spikes in HeLa cells by sensitizing the inositol 1,4,5-trisphosphate receptorThe Journal of Biological Chemistry 267:25113–25119.https://doi.org/10.1016/s0021-9258(19)74013-7
-
Zinc pyrithione induces ERK- and PKC-dependent necrosis distinct from TPEN-induced apoptosis in prostate cancer cellsBiochimica et Biophysica Acta 1823:544–557.https://doi.org/10.1016/j.bbamcr.2011.09.013
-
Egg-to-embryo transition is driven by differential responses to Ca(2+) oscillation numberDevelopmental Biology 250:280–291.https://doi.org/10.1006/dbio.2002.0788
-
Coordination of nuclear and cytoplasmic oocyte maturation in eutherian mammalsReproduction, Fertility, and Development 8:485–489.https://doi.org/10.1071/rd9960485
-
Stimulation of phospholipase C-epsilon by the M3 muscarinic acetylcholine receptor mediated by cyclic AMP and the GTPase Rap2BThe Journal of Biological Chemistry 277:16805–16813.https://doi.org/10.1074/jbc.M112024200
-
Differential distribution of inositol trisphosphate receptor isoforms in mouse oocytesBiology of Reproduction 60:49–57.https://doi.org/10.1095/biolreprod60.1.49
-
Detection and imaging of zinc secretion from pancreatic beta-cells using a new fluorescent zinc indicatorJournal of the American Chemical Society 124:776–778.https://doi.org/10.1021/ja011774y
-
Minimal requirements for calcium oscillations driven by the IP3 receptorThe EMBO Journal 16:3533–3543.https://doi.org/10.1093/emboj/16.12.3533
-
Three-dimensional rearrangements within inositol 1,4,5-trisphosphate receptor by calciumThe Journal of Biological Chemistry 278:52881–52889.https://doi.org/10.1074/jbc.M309743200
-
Zinc transporters as potential therapeutic targets: An updated reviewJournal of Pharmacological Sciences 148:221–228.https://doi.org/10.1016/j.jphs.2021.11.007
-
Biphasic Ca2+ dependence of inositol 1,4,5-trisphosphate-induced Ca release in smooth muscle cells of the guinea pig taenia caeciThe Journal of General Physiology 95:1103–1122.https://doi.org/10.1085/jgp.95.6.1103
-
Calcium modulation of inositol 1,4,5-trisphosphate-induced calcium release from neuroblastoma x glioma hybrid (NG108-15) microsomesThe Journal of Biological Chemistry 261:16414–16420.https://doi.org/10.1016/s0021-9258(18)66582-2
-
Redox regulation of type-I inositol trisphosphate receptors in intact mammalian cellsThe Journal of Biological Chemistry 293:17464–17476.https://doi.org/10.1074/jbc.RA118.005624
-
Acetylcholine induces Ca 2+ oscillations via m3/m4 muscarinic receptors in the mouse oocytePflugers Archiv European Journal of Physiology 447:321–327.https://doi.org/10.1007/s00424-003-1184-y
-
Zinc: the metal of lifeComprehensive Reviews in Food Science and Food Safety 13:358–376.https://doi.org/10.1111/1541-4337.12067
-
Zinc availability regulates exit from meiosis in maturing mammalian oocytesNature Chemical Biology 6:674–681.https://doi.org/10.1038/nchembio.419
-
A comprehensive overview of the complex world of the endo- and sarcoplasmic reticulum Ca2+-leak channelsBiochimica et Biophysica Acta. Molecular Cell Research 1868:119020.https://doi.org/10.1016/j.bbamcr.2021.119020
-
The biology and dynamics of mammalian cortical granulesReproductive Biology and Endocrinology 9:149.https://doi.org/10.1186/1477-7827-9-149
-
Coordination dynamics of zinc in proteinsChemical Reviews 109:4682–4707.https://doi.org/10.1021/cr800556u
-
TPEN induces apoptosis independently of zinc chelator activity in a model of acute lymphoblastic leukemia and ex vivo acute leukemia cells through oxidative stress and mitochondria caspase-3- and AIF-dependent pathwaysOxidative Medicine and Cellular Longevity 2012:313275.https://doi.org/10.1155/2012/313275
-
Dynamic zinc fluxes regulate meiotic progression in Caenorhabditis elegans†Biology of Reproduction 107:406–418.https://doi.org/10.1093/biolre/ioac064
-
Calcium signals for egg activation in mammalsJournal of Pharmacological Sciences 100:545–552.https://doi.org/10.1254/jphs.cpj06003x
-
Phospholipase Cζ binding to PtdIns(4,5)P2 requires the XY-linker regionJournal of Cell Science 124:2582–2590.https://doi.org/10.1242/jcs.083485
-
Essential role of the EF-hand domain in targeting sperm phospholipase Cζ to membrane phosphatidylinositol 4,5-Bisphosphate (PIP2)Journal of Biological Chemistry 290:29519–29530.https://doi.org/10.1074/jbc.M115.658443
-
A competitive chemical-proteomic platform to identify zinc-binding cysteinesACS Chemical Biology 9:258–265.https://doi.org/10.1021/cb400622q
-
Structural basis for the regulation of inositol trisphosphate receptors by Ca2+ and IP3Nature Structural & Molecular Biology 25:660–668.https://doi.org/10.1038/s41594-018-0089-6
-
Effects of Ca2+ chelators on purified inositol 1,4,5-trisphosphate (InsP3) receptors and InsP3-stimulated Ca2+ mobilizationThe Journal of Biological Chemistry 268:11528–11533.https://doi.org/10.1016/s0021-9258(19)50232-0
-
Complexes of 1-hydroxy 2-pyridinethioneJournal of Inorganic and Nuclear Chemistry 26:1277–1281.https://doi.org/10.1016/0022-1902(64)80210-4
-
PLCζ Induced Ca2+ oscillations in mouse eggs involve a positive feedback cycle of Ca2+ induced InsP3 formation from cytoplasmic PIP2Frontiers in Cell and Developmental Biology 6:1–14.https://doi.org/10.3389/fcell.2018.00036
-
Molecular basis of mammalian egg activationCurrent Topics in Developmental Biology 30:21–62.https://doi.org/10.1016/s0070-2153(08)60563-3
-
Metal ion fluxes controlling amphibian fertilizationNature Chemistry 13:683–691.https://doi.org/10.1038/s41557-021-00705-2
-
Molecular and functional evidence for multiple Ca2+-binding domains in the type 1 inositol 1,4,5-trisphosphate receptorThe Journal of Biological Chemistry 272:25899–25906.https://doi.org/10.1074/jbc.272.41.25899
-
Synaptic Zinc: an emerging player in parkinson’s diseaseInternational Journal of Molecular Sciences 22:1–16.https://doi.org/10.3390/ijms22094724
-
Modulators of calcium signalling at fertilizationOpen Biology 10:200118.https://doi.org/10.1098/rsob.200118
-
Comparative biology of calcium signaling during fertilization and egg activation in animalsDevelopmental Biology 211:157–176.https://doi.org/10.1006/dbio.1999.9340
-
Mechanism of Ca2+ release at fertilization in mammalsThe Journal of Experimental Zoology 285:267–275.https://doi.org/10.1002/(sici)1097-010x(19991015)285:3<267::aid-jez10>3.0.co;2-p
-
IP(3) receptors: toward understanding their activationCold Spring Harbor Perspectives in Biology 2:a004010.https://doi.org/10.1101/cshperspect.a004010
-
Structure of ionomycin - A novel diacidic polyether antibiotic having high affinity for calcium ionsJournal of the American Chemical Society 101:3344–3353.https://doi.org/10.1021/ja00506a035
-
Critical regions for activation gating of the inositol 1,4,5-trisphosphate receptorThe Journal of Biological Chemistry 278:16551–16560.https://doi.org/10.1074/jbc.M300646200
-
Regulation of inositol 1,4,5-trisphosphate receptor function during mouse oocyte maturationJournal of Cellular Physiology 227:705–717.https://doi.org/10.1002/jcp.22778
-
Regulation of endoplasmic reticulum Ca(2+) oscillations in mammalian eggsJournal of Cell Science 126:5714–5724.https://doi.org/10.1242/jcs.136549
-
Mitochondrial dynamics controlled by mitofusins define organelle positioning and movement during mouse oocyte maturationMolecular Human Reproduction 20:1090–1100.https://doi.org/10.1093/molehr/gau064
-
Ca2+ signaling and homeostasis in mammalian oocytes and eggsCold Spring Harbor Perspectives in Biology 11:a035162.https://doi.org/10.1101/cshperspect.a035162
-
Inositol 1,4,5-trisphosphate receptor ubiquitination is mediated by mammalian Ubc7, a component of the endoplasmic reticulum-associated degradation pathway, and is inhibited by chelation of intracellular Zn2+The Journal of Biological Chemistry 278:38238–38246.https://doi.org/10.1074/jbc.M305600200
-
Intracellular zinc modulates cardiac ryanodine receptor-mediated calcium releaseThe Journal of Biological Chemistry 290:17599–17610.https://doi.org/10.1074/jbc.M115.661280
-
Zinc is a novel intracellular second messengerThe Journal of Cell Biology 177:637–645.https://doi.org/10.1083/jcb.200702081
Article and author information
Author details
Funding
Japan Society for the Promotion of Science
- Hiroki Akizawa
Eunice Kennedy Shriver National Institute of Child Health and Human Development (RO1 HD092499)
- Rafael A Fissore
National Institute of Food and Agriculture (2021-06893)
- Rafael A Fissore
The funders had no role in study design, data collection and interpretation, or the decision to submit the work for publication.
Acknowledgements
We thank Ms. Changli He for technical support and Dr. James Chambers for support with confocal microscopy support. We thank all members of the Fissore lab for useful discussions and suggestions. We thank Jan B Parys, KU Leuven, Belgium, for initial discussions and advice.
Ethics
The University of Massachusetts Institutional Animal Care and Use Committee (IACUC) approved all animal experiments and protocols (#4650).
Version history
- Sent for peer review:
- Preprint posted:
- Reviewed Preprint version 1:
- Reviewed Preprint version 2:
- Version of Record published:
Cite all versions
You can cite all versions using the DOI https://doi.org/10.7554/eLife.88082. This DOI represents all versions, and will always resolve to the latest one.
Copyright
© 2023, Akizawa et al.
This article is distributed under the terms of the Creative Commons Attribution License, which permits unrestricted use and redistribution provided that the original author and source are credited.
Metrics
-
- 1,238
- views
-
- 137
- downloads
-
- 10
- citations
Views, downloads and citations are aggregated across all versions of this paper published by eLife.
Download links
Downloads (link to download the article as PDF)
Open citations (links to open the citations from this article in various online reference manager services)
Cite this article (links to download the citations from this article in formats compatible with various reference manager tools)
Further reading
-
- Cancer Biology
- Cell Biology
Testicular microcalcifications consist of hydroxyapatite and have been associated with an increased risk of testicular germ cell tumors (TGCTs) but are also found in benign cases such as loss-of-function variants in the phosphate transporter SLC34A2. Here, we show that fibroblast growth factor 23 (FGF23), a regulator of phosphate homeostasis, is expressed in testicular germ cell neoplasia in situ (GCNIS), embryonal carcinoma (EC), and human embryonic stem cells. FGF23 is not glycosylated in TGCTs and therefore cleaved into a C-terminal fragment which competitively antagonizes full-length FGF23. Here, Fgf23 knockout mice presented with marked calcifications in the epididymis, spermatogenic arrest, and focally germ cells expressing the osteoblast marker Osteocalcin (gene name: Bglap, protein name). Moreover, the frequent testicular microcalcifications in mice with no functional androgen receptor and lack of circulating gonadotropins are associated with lower Slc34a2 and higher Bglap/Slc34a1 (protein name: NPT2a) expression compared with wild-type mice. In accordance, human testicular specimens with microcalcifications also have lower SLC34A2 and a subpopulation of germ cells express phosphate transporter NPT2a, Osteocalcin, and RUNX2 highlighting aberrant local phosphate handling and expression of bone-specific proteins. Mineral disturbance in vitro using calcium or phosphate treatment induced deposition of calcium phosphate in a spermatogonial cell line and this effect was fully rescued by the mineralization inhibitor pyrophosphate. In conclusion, testicular microcalcifications arise secondary to local alterations in mineral homeostasis, which in combination with impaired Sertoli cell function and reduced levels of mineralization inhibitors due to high alkaline phosphatase activity in GCNIS and TGCTs facilitate osteogenic-like differentiation of testicular cells and deposition of hydroxyapatite.
-
- Cell Biology
G protein-coupled receptors (GPCRs) are integral membrane proteins which closely interact with their plasma membrane lipid microenvironment. Cholesterol is a lipid enriched at the plasma membrane with pivotal roles in the control of membrane fluidity and maintenance of membrane microarchitecture, directly impacting on GPCR stability, dynamics, and function. Cholesterol extraction from pancreatic beta cells has previously been shown to disrupt the internalisation, clustering, and cAMP responses of the glucagon-like peptide-1 receptor (GLP-1R), a class B1 GPCR with key roles in the control of blood glucose levels via the potentiation of insulin secretion in beta cells and weight reduction via the modulation of brain appetite control centres. Here, we unveil the detrimental effect of a high cholesterol diet on GLP-1R-dependent glucoregulation in vivo, and the improvement in GLP-1R function that a reduction in cholesterol synthesis using simvastatin exerts in pancreatic islets. We next identify and map sites of cholesterol high occupancy and residence time on active vs inactive GLP-1Rs using coarse-grained molecular dynamics (cgMD) simulations, followed by a screen of key residues selected from these sites and detailed analyses of the effects of mutating one of these, Val229, to alanine on GLP-1R-cholesterol interactions, plasma membrane behaviours, clustering, trafficking and signalling in INS-1 832/3 rat pancreatic beta cells and primary mouse islets, unveiling an improved insulin secretion profile for the V229A mutant receptor. This study (1) highlights the role of cholesterol in regulating GLP-1R responses in vivo; (2) provides a detailed map of GLP-1R - cholesterol binding sites in model membranes; (3) validates their functional relevance in beta cells; and (4) highlights their potential as locations for the rational design of novel allosteric modulators with the capacity to fine-tune GLP-1R responses.