Pharmacologic activation of integrated stress response kinases inhibits pathologic mitochondrial fragmentation
eLife Assessment
This important study identifies a new class of small molecules that activate the integrated stress response (ISR) via the kinase HRI. Convincing evidence, including the image analysis pipeline, indicates that two of these compounds promote mitochondrial elongation and protect against mitochondrial fragmentation caused by chemical stress conditions or by genetic alterations. These findings open an avenue for new strategies for mitochondrial dysfunction targeting linked to ISR alterations.
https://doi.org/10.7554/eLife.100541.3.sa0Important: Findings that have theoretical or practical implications beyond a single subfield
- Landmark
- Fundamental
- Important
- Valuable
- Useful
Convincing: Appropriate and validated methodology in line with current state-of-the-art
- Exceptional
- Compelling
- Convincing
- Solid
- Incomplete
- Inadequate
During the peer-review process the editor and reviewers write an eLife Assessment that summarises the significance of the findings reported in the article (on a scale ranging from landmark to useful) and the strength of the evidence (on a scale ranging from exceptional to inadequate). Learn more about eLife Assessments
Abstract
Excessive mitochondrial fragmentation is associated with the pathologic mitochondrial dysfunction implicated in the pathogenesis of etiologically diverse diseases, including many neurodegenerative disorders. The integrated stress response (ISR) – comprising the four eIF2α kinases PERK, GCN2, PKR, and HRI – is a prominent stress-responsive signaling pathway that regulates mitochondrial morphology and function in response to diverse types of pathologic insult. This suggests that pharmacologic activation of the ISR represents a potential strategy to mitigate pathologic mitochondrial fragmentation associated with human disease. Here, we show that pharmacologic activation of the ISR kinases HRI or GCN2 promotes adaptive mitochondrial elongation and prevents mitochondrial fragmentation induced by the calcium ionophore ionomycin. Further, we show that pharmacologic activation of the ISR reduces mitochondrial fragmentation and restores basal mitochondrial morphology in patient fibroblasts expressing the pathogenic D414V variant of the pro-fusion mitochondrial GTPase MFN2 associated with neurological dysfunctions, including ataxia, optic atrophy, and sensorineural hearing loss. These results identify pharmacologic activation of ISR kinases as a potential strategy to prevent pathologic mitochondrial fragmentation induced by disease-relevant chemical and genetic insults, further motivating the pursuit of highly selective ISR kinase-activating compounds as a therapeutic strategy to mitigate mitochondrial dysfunction implicated in diverse human diseases.
Introduction
The integrated stress response (ISR) comprises four stress-regulated kinases – PERK, PKR, GCN2, and HRI – that selectively phosphorylate eIF2α in response to diverse pathologic insults (Costa-Mattioli and Walter, 2020; Pakos-Zebrucka et al., 2016). The ISR has recently emerged as a prominent stress-responsive signaling pathway activated by different types of mitochondrial stress (Anderson and Haynes, 2020; Quirós et al., 2016; Winter et al., 2022). Mitochondrial uncoupling or inhibition of ATP synthase activates the ISR through a mechanism involving the cytosolic accumulation and oligomerization of the mitochondrial protein DELE1, which binds to and activates the ISR kinase HRI (Fessler et al., 2020; Fessler et al., 2022; Guo et al., 2020; Sekine et al., 2023; Yang et al., 2023a). Complex I inhibition can also activate the ISR downstream of the ISR kinase GCN2 (Mick et al., 2020). Further, CRISPRi-depletion of mitochondrial proteostasis factors preferentially activates the ISR over other stress-responsive signaling pathways (Madrazo et al., 2024). These results identify the ISR as an important stress-responsive signaling pathway activated in response to many different mitochondrial insults.
Consistent with its activation by mitochondrial stress, the ISR regulates many aspects of mitochondrial biology. ISR kinases are activated in response to stress through a conserved mechanism involving dimerization and autophosphorylation (Costa-Mattioli and Walter, 2020; Pakos-Zebrucka et al., 2016). Once activated, these kinases phosphorylate eIF2α, resulting in both a transient attenuation of new protein synthesis and the activation of stress-responsive transcription factors such as ATF4 (Costa-Mattioli and Walter, 2020; Pakos-Zebrucka et al., 2016). The ISR promotes adaptive remodeling of mitochondrial pathways through both transcriptional and translational signaling. For example, the activation of ATF4 downstream of phosphorylated eIF2α transcriptionally regulates the expression of mitochondrial chaperones (e.g., the mitochondrial HSP70 HSPA9) and proteases (e.g., the AAA+ protease LONP1), increasing mitochondrial proteostasis capacity during stress (Hori et al., 2002; Han et al., 2013). ISR-dependent translational attenuation also enhances mitochondrial proteostasis through the selective degradation of the core import subunit TIM17A, which slows mitochondrial protein import and reduces the load of newly synthesized proteins entering mitochondria during stress (Rainbolt et al., 2013). Apart from proteostasis, transcriptional and translational signaling induced by the activation of ISR kinases also regulates many other mitochondrial functions, including phospholipid synthesis, cristae organization, and electron transport chain activity (Balsa et al., 2019; Barad et al., 2023; Latorre-Muro et al., 2021; Lebeau et al., 2018; Perea et al., 2023b).
Intriguingly, the morphology of mitochondria is also regulated by ISR signaling. Mitochondrial morphology is dictated by the relative activities of pro-fission and pro-fusion GTPases localized to the outer and inner mitochondrial membranes (OMM and IMM, respectively) (Chan, 2020; Quintana-Cabrera and Scorrano, 2023). These include the pro-fusion GTPases MFN1 and MFN2 and the pro-fission GTPase DRP1, all localized to the OMM. Previous results showed that ER stress promotes adaptive mitochondrial elongation downstream of the PERK arm of the ISR through a mechanism involving the accumulation of the phospholipid phosphatidic acid on the OMM where it inhibits the pro-fission GTPase DRP1 (Lebeau et al., 2018; Perea et al., 2023b; Perea et al., 2023a). This PERK-dependent increase of mitochondrial elongation functions to protect mitochondria during ER stress through multiple mechanisms, including enhanced regulation of respiratory chain activity, suppression of mitochondrial fragmentation, and reductions in the turnover of mitochondria by mitophagy (Lebeau et al., 2018; Perea et al., 2023b; Perea et al., 2023a). Apart from PERK, pharmacologic activation of the alternative ISR kinase GCN2 also promotes adaptive mitochondrial elongation (Perea et al., 2023a). This indicates that mitochondrial elongation may be a protective mechanism that can be pharmacologically accessed by activating multiple different ISR kinases.
Excessive mitochondrial fragmentation is a pathologic hallmark of numerous human diseases, including several neurodegenerative disorders (Chan, 2020; Sharma et al., 2021a; Chen et al., 2023; Liu et al., 2020; Sprenger and Langer, 2019). Fragmented mitochondria are often associated with mitochondrial dysfunctions, including impaired respiratory chain activity and dysregulation of mitophagy (Chan, 2020; Sharma et al., 2021a; Chen et al., 2023; Liu et al., 2020; Sprenger and Langer, 2019; Giacomello et al., 2020; Wai and Langer, 2016). Thus, pathologic increases in mitochondrial fragmentation are linked to many mitochondrial dysfunctions implicated in human disease. Consistent with this observation, interventions that prevent mitochondrial fragmentation have been shown to correct pathologic mitochondrial dysfunction in models of many different diseases (Whitley et al., 2019; Bhatti et al., 2023). Notably, genetic or pharmacologic inhibition of the pro-fission GTPase DRP1 blocks pathologic mitochondrial fragmentation and subsequent mitochondrial dysfunction in cellular and in vivo models of diverse neurodegenerative diseases, including Charcot–Marie–Tooth (CMT) Type 2A and Type 2B, spastic paraplegia, optic atrophy, Huntington’s disease, amyotrophic lateral sclerosis, and Alzheimer’s disease (Gu et al., 2022; Das et al., 2022; Chen et al., 2022; Yang et al., 2023b; Guo et al., 2013; Joshi et al., 2018; Bera et al., 2022; Manczak et al., 2016; Kandimalla et al., 2022; Yan et al., 2015; Baek et al., 2017; Mou and Li, 2019).
The ability of ISR activation to promote mitochondrial elongation suggests that pharmacologic activation of ISR kinases could mitigate the pathologic mitochondrial fragmentation implicated in etiologically diverse human diseases. To test this idea, we probed the potential for pharmacologic activation of different ISR kinases to reduce mitochondrial fragmentation induced by disease-relevant chemical and genetic insults. Previous work identified the small-molecule halofuginone as a potent activator of the ISR kinase GCN2 that promotes ISR-dependent, adaptive mitochondrial elongation (Perea et al., 2023a; Keller et al., 2012). However, few other compounds were available that selectively activated other ISR kinases and were suitable for probing mitochondrial adaptation induced by ISR kinase activation (Perea et al., 2023a). Here, we used a small-molecule screening platform to identify two nucleoside mimetic compounds, 0357 and 3610, that preferentially activate the ISR downstream of the ISR kinase HRI. Using these compounds, we demonstrate that pharmacologic HRI activators also promote adaptive, ISR-dependent mitochondrial elongation. We go on to show that pharmacologic activation of the ISR with these compounds prevents DRP1-mediated mitochondrial fragmentation induced by the calcium ionophore ionomycin (Ji et al., 2015; Ji et al., 2017). Further, we show that compound-dependent activation of the ISR reduces the population of fragmented mitochondria and rescues basal mitochondrial network morphology in patient fibroblasts expressing the D414V variant of the pro-fusion GTPase MFN2 associated with a complex clinical phenotype including ataxia, optic atrophy, and sensorineural hearing loss (Sharma et al., 2021b). These results demonstrate the potential for pharmacologic activation of the ISR to prevent pathologic mitochondrial fragmentation in disease-relevant models. Moreover, our work further motivates the continued development of highly selective activators of ISR kinases as a potential therapeutic strategy to mitigate the pathologic mitochondrial dysfunction implicated in etiologically diverse human diseases.
Results
The nucleoside mimetic compounds 0357 and 3610 preferentially activate the ISR downstream of HRI
The small-molecule halofuginone activates the ISR kinase GCN2 and induces ISR-dependent mitochondrial elongation (Perea et al., 2023a; Keller et al., 2012) However, few other compounds are available that selectively activate other ISR kinases through mechanisms that allow for ISR-dependent mitochondrial remodeling (Perea et al., 2023a). For example, BtdCPU activates the ISR kinase HRI through a mechanism involving mitochondrial uncoupling, precluding its use for probing ISR-dependent protection of mitochondria (Perea et al., 2023a). To address this limitation and define the potential for pharmacologic activation of other ISR kinases to promote adaptive mitochondrial elongation, we established and implemented a screening platform to identify compounds that activated ISR signaling downstream of alternative ISR kinases (Figure 1A). In this screen, we used the ATF4-FLuc translational reporter of the ISR (Figure 1—figure supplement 1A; Yang et al., 2023a) We confirmed that ISR-activating stressors including the ER stressor thapsigargin (Tg) and the ATP synthase inhibitor oligomycin A (OA) robustly activated this reporter (Figure 1—figure supplement 1B). We then used this reporter to screen the ~3k nucleoside mimetic analog compound library (10 µM) and monitored ATF4-FLuc activity 8 hr after treatment. Our primary screen identified 34 hit compounds that activated the ATF4-FLuc reporter with a robust Z-score of greater than 3 fold. We then removed highly reactive compounds and pan-assay interference compounds (PAINS), which reduced the number of hits to 9 (Figure 1B). These compounds were re-purchased and then tested in dose response for ATF4-FLuc activation (Figure 1—figure supplement 1C). This identified compounds 0357 and 3610 as the compounds that most efficaciously activated the ATF4-FLuc reporter, albeit with low potency (EC50 > 10 µM). We confirmed that co-treatment with the highly selective ISR inhibitor ISRIB (Sidrauski et al., 2013; Zyryanova et al., 2018) blocked ATF4-FLuc activation induced by these compounds, confirming this activation can be attributed to the ISR (Figure 1C and D). Further, we used qPCR to show that treatment with 0357 or 3610 increased expression of the ISR target genes ASNS and CHAC1 in HEK293 and MEF cells (Figure 1—figure supplement 1D and E; Kreß et al., 2023; Grandjean et al., 2019). Importantly, these compounds did not activate luciferase reporters of other stress-responsive signaling pathway such as the unfolded protein response (UPR; XBP1-RLuc) (Grandjean et al., 2020; Plate et al., 2016), the heat shock response (HSR; HSE-FLuc) (Calamini et al., 2011), or the oxidative stress response (OSR; ARE-FLuc) (Ibrahim et al., 2020; Figure 1E and F). Further, treatment with these compounds did not induce expression of the UPR target gene BiP, the HSR target gene HSPA1A, or the OSR target gene NQO1 in HEK293 cells (Figure 1—figure supplement 1F). Finally, treatment with 0357 or 3610 did not significantly reduce viability of HEK293 cells (Figure 1—figure supplement 1G). These results indicate compounds 0357 and 3610 preferentially activate the ISR compared to other stress-responsive signaling pathways.
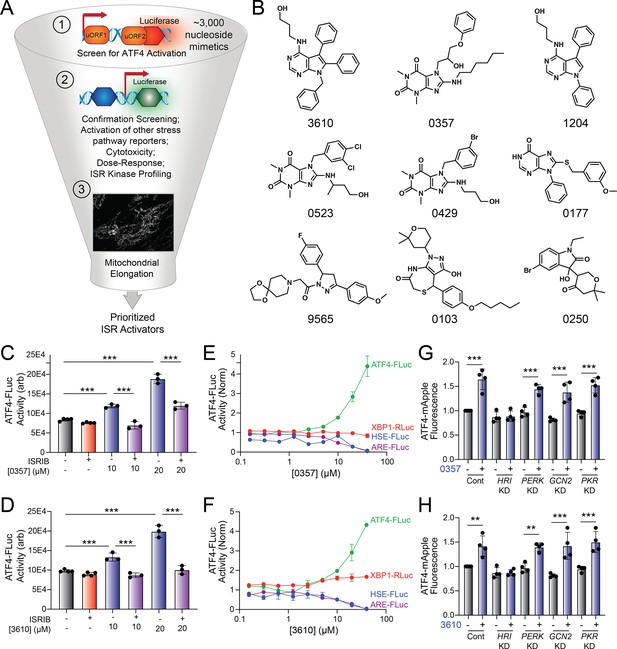
Identification of nucleoside mimetics that preferentially activate the integrated stress response (ISR) kinase HRI.
(A) Screening pipeline used to identify selective ISR kinase-activating compounds that promote protective mitochondrial elongation. (B) Structures of the top 9 ISR-activating compounds identified in our nucleoside mimetic screen. (C, D) ATF4-FLuc activity in HEK293 cells stably expressing ATF4-FLuc (Yang et al., 2023a) treated for 8 hr with the indicated concentration of 0357 (C) or 3610 (D) in the absence or presence of ISRIB (200 nM). (E, F) Activation of the ATF4-FLuc ISR translational reporter (green), the XBP1-RLuc UPR reporter (red), the HSE-FLuc HSR reporter (blue), or the ARE-FLuc OSR reporter (purple) stably expressed in HEK293 cells treated with the indicated concentration of 0357 (E) or 3610 (F) for 16 hr. (G, H) ATF4-mAPPLE fluorescence in HEK293 cells stably expressing ATF4-mAPPLE and CRISPRi depleted of the indicated ISR kinase (Guo et al., 2020) treated for 8 hr with 0357 (G, 20 µM) or 3610 (H, 20 µM). **p<0.01, ***p<0.005 for one-way ANOVA.
-
Figure 1—source data 1
Excel spreadsheet containing source data for panels C-H.
- https://cdn.elifesciences.org/articles/100541/elife-100541-fig1-data1-v1.xlsx
Next, we sought to identify the specific ISR kinase responsible for ISR activation induced by these two nucleoside mimetics. We monitored the compound-dependent activation of an ATF4-mAPPLE fluorescent reporter stably expressed in HEK293 cells CRISPRi-depleted of each individual ISR kinase (Figure 1—figure supplement 1A; Guo et al., 2020; Perea et al., 2023a). We previously used this assay to confirm that halofuginone activates the ISR downstream of GCN2 and BtdCPU activates the ISR downstream of HRI (Perea et al., 2023a). Treatment with either 0357 or 3610 activates the ATF4-mAPPLE reporter in control cells (Figure 1G and H). CRISPRi-depletion of HRI, but no other ISR kinase, blocked ATF4-FLuc activation induced by these two compounds. This finding indicates that these compounds activate the ISR downstream of HRI. Collectively, these results identify 0357 and 3610 as nucleoside mimetic compounds that preferentially activate the ISR through a mechanism involving the ISR kinase HRI.
Pharmacologic HRI activators promote mitochondrial elongation
Halofuginone-dependent activation of GCN2 promotes adaptive mitochondrial elongation (Perea et al., 2023a). However, it is currently unclear if pharmacologic activation of other ISR kinases can similarly induce mitochondrial elongation. Here, we tested the ability of our HRI-activating compounds 0357 and 3610 to induce mitochondrial elongation downstream of the ISR. Previously, ISR-dependent mitochondrial elongation was quantified by manually classifying cells as containing fragmented, tubular, or elongated networks (Lebeau et al., 2018; Perea et al., 2023b). However, since 0357 and 3610 activate ISR signaling to lower levels than that observed for other compounds (e.g., halofuginone), we posited that these compounds may induce more modest mitochondrial elongation that may be difficult to quantify using this manual approach. To address this, we implemented an automated image analysis pipeline using Imaris software to quantify mitochondrial elongation in MEF cells stably expressing mitochondrial-targeted GFP (mtGFP) treated with our compounds (Figure 2—figure supplement 1A); (Wang et al., 2012). We collected Z-stack confocal images of mtGFP-expressing MEF cells (MEFmtGFP) and processed images using a deconvolution filter in FIJI to reduce the background and enhance the fluorescent signal. We used the ‘Surfaces’ module on Imaris to generate three-dimensional (3D) segmentation models of mitochondria visible in the deconvolved Z-stacks. Using the surfaces module, we quantified parameters defining mitochondrial shape, including bounding box length, sphericity, and ellipsoid principal axis length (Figure 2—figure supplement 1A). Treatment with conditions that induce mitochondrial elongation, such as the ER stressor thapsigargin (Tg) and the GCN2 activator halofuginone (HF), increased bounding box and ellipsoid principal axis length, while reducing sphericity (Figure 2A–D, Figure 2—figure supplement 1B–D) – all changes consistent with increases in mitochondrial length. In contrast, treatment with conditions that promote mitochondrial fragmentation, such as the mitochondrial uncouplers BtdCPU and CCCP (both compounds that activate HRI) (Fessler et al., 2020; Perea et al., 2023a), reduced bounding box length and ellipsoid principal axis length, while increasing sphericity (Figure 2A–D, Figure 2—figure supplement 1B–D) – all changes consistent with increased mitochondrial fragmentation.
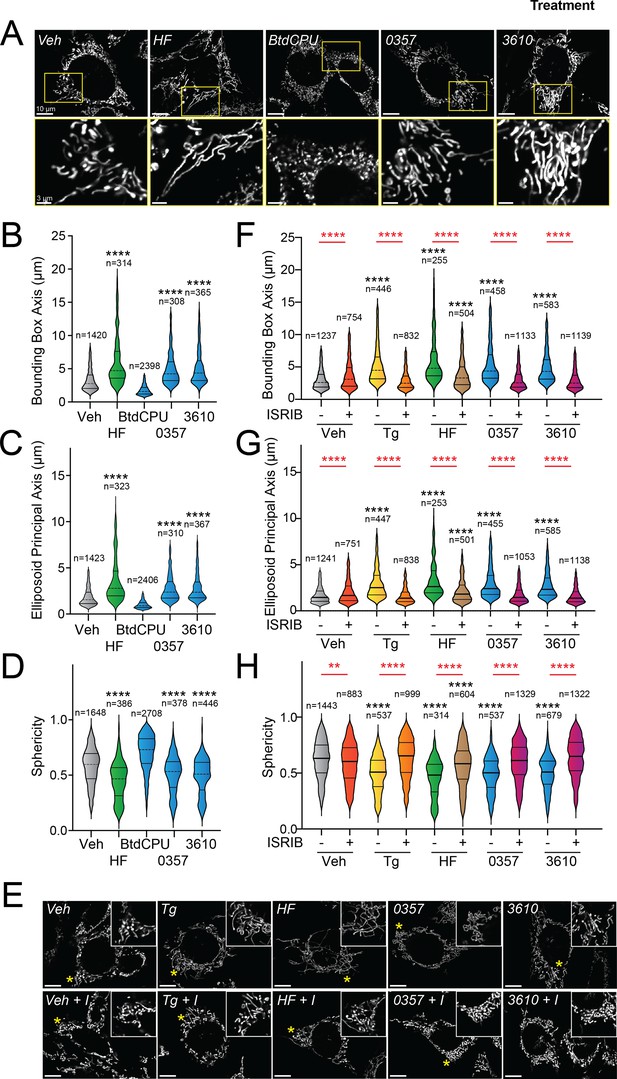
Pharmacologic HRI activation induces integrated stress response (ISR)-dependent mitochondrial elongation.
(A) Representative images of MEF cells stably expressing mtGFP (MEFmtGFP) (Wang et al., 2012) treated for 6 hr with vehicle (veh), halofuginone (HF, 100 nM), BtdCPU (10 µM), 0357 (10 µM), or 3610 (10 µM). The inset shows a 3-fold magnification of the region indicated by the yellow box. Scale bars, 10 µm (top) and 3.33 µm (bottom). (B–D) Quantification of bounding box axis, ellipsoid principal axis, and sphericity from the entire dataset of representative images shown in (A). The number of individual measurements for each condition is shown above. (E) Representative images of MEFmtGFP cells treated for 6 hr with vehicle (veh), thapsigargin (Tg; 0.5 µM) halofuginone (HF, 100 nM), 0357 (10 µM), or 3610 (10 µM) in the presence or absence of ISRIB (200 nM). The inset shows 3-fold magnification of the image centered on the asterisks. Scale bars, 10 µm. (F–H) Quantification of bounding box axis, ellipsoid principal axis, and sphericity from the entire dataset of representative images shown in (E). The number of 3D segmentations used for the individual measurements for each condition is shown above. *p<0.05, ****p<0.001 for Kruskal–Wallis ANOVA. Black asterisks indicate comparison to vehicle-treated cells. Red asterisks show comparisons for ISRIB co-treatment.
-
Figure 2—source data 1
Excel spreadsheet containing source data for panels B-D and F-H.
- https://cdn.elifesciences.org/articles/100541/elife-100541-fig2-data1-v1.xlsx
We next applied this approach to define the impact of our pharmacologic HRI activators on mitochondrial morphology. Treatment with either 0357 or 3610 for 6 hr increased both the bounding box and ellipsoid principal axis length, while decreasing mitochondrial sphericity (Figure 2A–D). This result indicates that both these compounds induced mitochondrial elongation. Co-treatment with the selective ISR inhibitor ISRIB blocked these changes in mitochondria shape, indicating that these compounds induce mitochondrial elongation through an ISR-dependent mechanism (Figure 2E–H). ISRIB co-treatment also blocked mitochondrial elongation induced by the ER stressor thapsigargin (Tg) and the GCN2 activator halofuginone (HF), as predicted (Lebeau et al., 2018; Perea et al., 2023a). These results indicate that, like halofuginone, pharmacologic HRI activators also induce adaptive, ISR-dependent mitochondrial elongation. Further, these results suggest that the pharmacologic activation of different ISR kinases can induce protective elongation of mitochondria in the absence of cellular stress.
Pharmacologic ISR activation suppresses ionomycin-induced mitochondrial fragmentation
The ability for our pharmacologic GCN2 or HRI activators to induce adaptive mitochondrial elongation suggests that enhancing signaling through these kinases may suppress mitochondrial fragmentation induced by pathologic insults such as calcium dysregulation (Calvo-Rodriguez and Bacskai, 2020; Calvo-Rodriguez et al., 2020; Garbincius and Elrod, 2022; Matuz-Mares et al., 2022). Treatment with the calcium ionophore ionomycin induces rapid, DRP1-dependent mitochondrial fragmentation in cell culture models (Ji et al., 2015; Ji et al., 2017). We pretreated MEFmtGFP cells for 6 hr with the GCN2 activator halofuginone or our two HRI-activating compounds (0357 and 3610) and subsequently challenged these cells with ionomycin. We then monitored mitochondrial morphology over a 15 min timecourse. As expected, ionomycin rapidly increased the accumulation of fragmented mitochondria in these cells, evidenced by reductions in both bounding box and ellipsoid principal axis length and increases of organelle sphericity (Figure 3—figure supplement 1A–D). Pretreatment with the ER stressor thapsigargin, which promotes stress-induced mitochondrial elongation downstream of the PERK ISR kinase, reduced the accumulation of fragmented mitochondria in ionomycin-treated cells (Figure 3A–D), as previously reported (Perea et al., 2023b). Intriguingly, treatment with halofuginone, 0357, or 3610 also reduced the accumulation of fragmented mitochondria in ionomycin-treated cells. Instead, mitochondria in cells pretreated with these ISR kinase activators and challenged with ionomycin demonstrated mitochondrial lengths and sphericity similar to that observed in vehicle-treated MEFmtGFP cells (Figure 3A–D). These results indicate that pharmacologic activation of different ISR kinases can suppress the accumulation of fragmented mitochondria following ionomycin-induced calcium dysregulation.

Pharmacologic activation of integrated stress response (ISR) kinases prevents ionomycin-dependent accumulation of fragmented mitochondria.
(A) Representative images of MEFmtGFP cells pretreated for 6 hr with vehicle (veh), thapsigargin (Tg, 500 nM), halofuginone (HF, 100 nM), 0357 (10 µM), or 3610 (10 µM) and then challenged with ionomycin (1 µM) for the indicated time. The inset shows 3-fold magnification of the image centered on the asterisk. Scale bars, 10 µm. (B–D) Quantification of bounding box axis length, ellipsoid principal axis length, and sphericity from the entire dataset of representative images shown in (A). The black dashed line shows the mean value of vehicle-treated cells prior to ionomycin treatment. The dashed red line shows the mean value of vehicle-treated cells following 15 min treatment with ionomycin. The number of 3D segmentations used for the individual measurements for each condition is shown above. *p<0.05, **p<0.01, ***p<0.005, ****p<0.001 for Kruskal–Wallis ANOVA. Black asterisks show comparison with vehicle-treated cells.
-
Figure 3—source data 1
Excel spreadsheet containing source data for panels B-D.
- https://cdn.elifesciences.org/articles/100541/elife-100541-fig3-data1-v1.xlsx
Pharmacologic activation of ISR kinases restores basal mitochondrial morphology in patient fibroblasts expressing disease-associated MFN2D414V
Over 150 pathogenic variants in the pro-fusion GTPase MFN2 are causatively associated with the autosomal-dominant peripheral neuropathy CMT Type 2A (Cartoni and Martinou, 2009; Zaman and Shutt, 2022; Alberti et al., 2024). While these pathogenic variants can impact diverse aspects of mitochondrial biology (Zaman and Shutt, 2022), many, including D414V, lead to increases in mitochondrial fragmentation (Sharma et al., 2021b; Cartoni and Martinou, 2009; Zaman and Shutt, 2022; Alberti et al., 2024). This can be attributed to reduced activity of MFN2-dependent fusion associated with these variants and a subsequent relative increase of DRP1-dependent mitochondrial fission. We predicted that pharmacologic activation of ISR kinases could rescue mitochondrial network morphology in patient fibroblasts expressing the disease-associated MFN2 variant D414V (MFN2D414V). To test this, we treated wild-type human fibroblasts and patient fibroblasts expressing MFN2D414V with halofuginone or our two HRI-activating compounds 0357 and 3610 and monitored mitochondrial network morphology by staining with MitoTracker. As reported previously, MFN2D414V-expressing fibroblasts showed shorter, more fragmented mitochondrial networks compared to control fibroblasts, reflected by reductions in both bounding box and ellipsoid principle axis lengths and increased sphericity (Figure 4A and E, Figure 4—figure supplement 1A–C; Sharma et al., 2021b). Treatment with halofuginone, 0357, or 3610 increased mitochondrial length in control fibroblasts (Figure 4A–D). These changes were inhibited by co-treatment with ISRIB, confirming these effects can be attributed to ISR activation. Intriguingly, all three compounds also increased mitochondrial length and reduced sphericity in MFN2D414V-expressing patient fibroblasts to levels similar to those observed in control fibroblasts, with halofuginone showing the largest effect (Figure 4E–H). Again, this increase in mitochondrial elongation was reversed by co-treatment with ISRIB. These results show that pharmacologic activation of different ISR kinases can rescue basal mitochondrial morphology in patient fibroblasts expressing the disease-associated MFN2D414V variant that causes dysregulation in various neurological functions, including ataxia, optic atrophy, and sensorineural hearing loss.
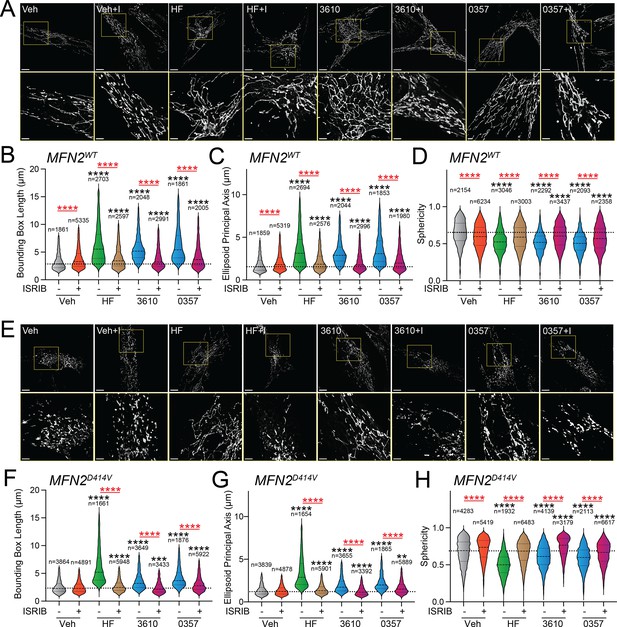
Pharmacologic activation of integrated stress response (ISR) kinases rescues basal mitochondrial morphology in patient fibroblasts expressing the disease-associated D414V MFN2 variant.
(A) Representative images of control human fibroblasts expressing MFN2WT treated for 6 hr with vehicle (veh), halofuginone (HF, 100 nM), 3610 (10 µM), 0357 (10 µM), and/or ISRIB (200 nM). The inset shows 3-fold magnification of the image centered on the asterisks. Scale bars, 15 µm (top) and 5 µM (bottom). (B–D) Quantification of bounding box axis (B), ellipsoid principal axis (C), and sphericity (D) from the entire dataset of images described in panel (A). The number of individual measurements for each condition is shown above. (E) Representative images of patient fibroblasts expressing MFN2D414V treated for 6 hr with veh, HF (100 nM), 3610 (10 µM), 0357 (10 µM), and/or ISRIB (200 nM). The inset shows 3-fold magnification of the image centered on the asterisks. Scale bars, 15 µm (top) and 5 µM (bottom). (F–H) Quantification of bounding box axis (F), ellipsoid principal axis (G), and sphericity (H) from the entire dataset of images described in panel (E). The number of 3D segmentations used for the individual measurements for each condition is shown above. *p<0.05, ***p<0.005, ****p<0.001 for Kruskal–Wallis ANOVA. Black asterisks show comparison with vehicle-treated cells. Red asterisks show comparisons for ISRIB co-treatment.
-
Figure 4—source data 1
Excel spreadsheet containing source data for panels B-D and F-H.
- https://cdn.elifesciences.org/articles/100541/elife-100541-fig4-data1-v1.xlsx
Discussion
Here, we show that pharmacologic activation of different ISR kinases can prevent mitochondrial fragmentation induced by disease-relevant chemical or genetic insults. We identify two nucleoside mimetic compounds that preferentially activate the ISR downstream of the ISR kinase HRI. We demonstrate that these two HRI activators promote adaptive, ISR-dependent mitochondrial elongation. Further, we show that treatment with these HRI-activating compounds or the GCN2 activator halofuginone prevents the accumulation of fragmented mitochondria induced by Ca2+ dysregulation and rescues mitochondrial network morphology in patient fibroblasts expressing the disease-associated, pathogenic D414V variant of MFN2. Collectively, these results demonstrate that pharmacologic activation of different ISR kinases can mitigate pathologic mitochondrial fragmentation induced by diverse disease-associated pathologic insults.
Mitochondrial fragmentation is often induced through a mechanism involving stress-dependent increases in the activity of the pro-fission GTPase DRP1 (Chan, 2020; Giacomello et al., 2020). This has motivated efforts to identify pharmacologic inhibitors of DRP1 to prevent mitochondrial fragmentation and subsequent organelle dysfunction associated with human disease (Bhatti et al., 2023; Bera et al., 2022; Yu et al., 2023). Activation of the ISR kinase PERK during ER stress promotes mitochondrial elongation through a mechanism involving inhibition of DRP1 (Perea et al., 2023b). This suggested that pharmacologic activation of other ISR kinases could also potentially inhibit DRP1 and block pathologic mitochondrial dysfunction induced by increased DRP1 activity. Consistent with this idea, we show that pharmacologic activation of the ISR using both GCN2 and HRI-activating compounds blocks the accumulation of fragmented mitochondria in ionomycin-treated cells – a condition that promotes mitochondrial fragmentation through increased DRP1-dependent fission (Ji et al., 2015; Ji et al., 2017). These results support a model whereby pharmacologic activation of different ISR kinases promotes organelle elongation by inhibiting DRP1 and suggests that pharmacologic activation of ISR kinases can be broadly applied to quell mitochondrial fragmentation in the myriad diseases associated with overactive DRP1.
Pathogenic variants in mitochondrial-targeted proteins are causatively associated with the onset and pathogenesis of numerous neurodegenerative disorders, including CMT, spinocerebellar ataxia (SCA), and spastic paraplegia (Chan, 2020; Sharma et al., 2021a; Gorman et al., 2016; Lightowlers et al., 2015; Song et al., 2021; Deshwal et al., 2020). In many of these diseases, mitochondrial fragmentation is linked to pathologic mitochondrial dysfunctions, such as reduced respiratory chain activity, decreased mtDNA, and increased apoptotic signaling. Intriguingly, pharmacologic or genetic inhibition of mitochondrial fragmentation mitigates cellular and mitochondrial pathologies associated with many of these diseases, highlighting the critical link between mitochondrial fragmentation and disease pathogenesis (Das et al., 2022; Chen et al., 2022; Yang et al., 2023b; Mou and Li, 2019). Herein, we show that pharmacologic activation of the ISR mitigates mitochondrial fragmentation in patient fibroblasts homozygous for the disease-associated D414V MFN2 variant. This finding highlights the potential for pharmacologic ISR activation to restore basal mitochondrial network morphology in cells expressing pathogenic MFN2 variants, although further study is necessary to determine the impact of ISR activation in cells from heterozygous patients expressing pathogenic MFN2 variants associated with CMT2A, the most common clinical phenotype associated with this disease. Regardless, our results are of substantial interest because over 70% of cases of axonal CMT are associated with pathogenic MFN2 variants, and to date, no disease-modifying therapies are available for any genetic subtype of CMT (Yoshimura et al., 2019; Pisciotta et al., 2021). Apart from MFN2 variants, our results also highlight the potential for pharmacologic ISR kinase activation to broadly mitigate pathologic mitochondrial fragmentation associated with the expression of disease-related, pathogenic variants of other mitochondrial proteins, which we are continuing to explore.
Apart from morphology, ISR signaling also promotes adaptive remodeling of many other aspects of mitochondrial biology, including proteostasis, electron transport chain activity, phospholipid synthesis, and apoptotic signaling (Hori et al., 2002; Han et al., 2013; Rainbolt et al., 2013; Balsa et al., 2019; Barad et al., 2023; Latorre-Muro et al., 2021; Lebeau et al., 2018; Perea et al., 2023b). While we specifically focus on defining the potential for pharmacologic activation of ISR kinases to rescue mitochondrial morphology in disease models, the potential of ISR activation to promote adaptive remodeling of other mitochondrial functions suggests that pharmacologic activators of ISR kinases could more broadly influence cellular and mitochondrial biology in disease states through the adaptive remodeling of these other pathways. For example, we previously showed that halofuginone-dependent GCN2 activation restored cellular ER stress sensitivity and mitochondrial electron transport chain activity in cells deficient in the alternative ISR kinase PERK (Perea et al., 2023a) – a model of neurodegenerative diseases associated with reduced PERK activity such as PSP and AD (Park et al., 2023; Yuan et al., 2018). Compounds that activate other ISR kinases are similarly predicted to promote the direct remodeling of mitochondrial pathways to influence these and other functions. Thus, as we, and others, continue defining the impact of pharmacologic ISR kinase activation on mitochondrial function, we predict to continue revealing new ways in which activation of ISR kinases directly regulates many other aspects of mitochondrial function disrupted in human disease.
Several different compounds have previously been reported to activate specific ISR kinases (Perea et al., 2023a; Ganz et al., 2020; Stockwell et al., 2012; Chen et al., 2011; Szaruga et al., 2023; Carlson et al., 2023; Thomson et al., 2024). However, the potential for many of these compounds to mitigate mitochondrial dysfunction in human disease is limited by factors including lack of selectivity for the ISR or a specific ISR kinase, off-target activities, or low therapeutic windows (Perea et al., 2023a). For example, the HRI activator BtdCPU activates the ISR through a mechanism involving mitochondrial uncoupling and subsequent mitochondrial fragmentation (Perea et al., 2023a). Here, we identify two nucleoside mimetic compounds that activate the ISR downstream of HRI and show selectivity for the ISR relative to other stress-responsive signaling pathways, providing new tools to probe mitochondrial remodeling induced by ISR kinase activation. However, the low potency of these compounds limits their translational potential to mitigate mitochondrial dysfunction in disease, necessitating the identification of new, highly selective ISR kinase-activating compounds. As we and others continue identifying next-generation ISR kinase activators with improved translational potential, it will be important to optimize the pharmacokinetics (PK) and pharmacodynamic (PD) profiles of these compounds to selectively enhance adaptive, protective ISR signaling in disease-relevant tissues, independent of maladaptive ISR signaling often associated with chronic, stress-dependent activation of this pathway (Costa-Mattioli and Walter, 2020; Pakos-Zebrucka et al., 2016). As described previously for activators the IRE1 arm of the UPR, such improvements can be achieved by defining optimized compound properties and dosing regimens to control the timing and extent of pathway activity (Madhavan et al., 2022). Thus, as we and others continue pursuing pharmacologic ISR kinase activation as a strategy to target mitochondrial dysfunction in disease, we anticipate that we will continue to learn more about the central role for this pathway in adapting mitochondria during stress and establish pharmacologic ISR kinase activation as a viable approach to treat mitochondrial dysfunction associated with etiologically diverse diseases.
Materials and methods
Reagent type (species) or resource | Designation | Source or reference | Identifiers | Additional information |
---|---|---|---|---|
Cell line (human) | HEK293 | ATCC | ||
Cell line (human) | HEK293 cells expressing XBP1-RLuc | Wiseman Lab (TSRI) | Grandjean et al., 2020; Plate et al., 2016 | |
Cell line (human) | HEK293 cells expressing ATF4-FLuc | Martin Kampmann’s lab (UCSF) | Yang et al., 2023a | |
Cell line (human) | HEK293 cells expressing ATF4-mAPPLE and CRISPRi-depleted of PERK, GCN2, HRI, or PKR | Martin Kampmann’s lab (UCSF) | Guo et al., 2020 | |
Cell line (mouse) | MEF cells stably expressing mitochondrial-targeted GFP (MEFmtGFP) | Peter Schultz’s lab (TSRI) | Wang et al., 2012 | |
Cell line (human) | Primary fibroblasts from patients expressing WT or D414V MFN2 | University of Calgary | Sharma et al., 2021a; Martens et al., 2020 | |
Commercial assay or kit | Promega Bright-Glo substrate | Promega | ||
Commercial assay or kit | Quick-RNA MiniPrepKit | Zymo Research | ||
Commercial assay or kit | High-Capacity Reverse Transcription Kit | Applied Biosystems | ||
Commercial assay or kit | Power SYBR Green PCR Master Mix | Applied Biosystems | ||
Chemical compound, drug | Thapsigargin (Tg) | Fisher Scientific | 50-464-294 | |
Chemical compound, drug | ISRIB | Sigma | SML0843 | |
Chemical compound, drug | CCCP | Sigma | C2759 | |
Chemical compound, drug | BtdCPU | Fisher | 32-489-210MG | |
Chemical compound, drug | Halofuginone (HF) | Sigma | 50-576-3001 | |
Chemical compound, drug | Oligomycin A | Selleck | S1478 | |
Chemical compound, drug | MitoTracker Green | Life Technologies | M7514 | |
Software, algorithm | Imaris 10.0 | Oxford Instruments | 3-D Surface Rendering Module |
Mammalian cell culture
Request a detailed protocolHEK293 cells (purchased from ATCC), HEK293 cells stably expressing XBP1-RLuc (Grandjean et al., 2020; Plate et al., 2016), HEK293 cells stably expressing HSE-FLuc, HEK293 cells stably expressing ATF4-FLuc (Yang et al., 2023a), HEK293 cells stably expressing ATF4-mAPPLE (a kind gift from Martin Kampmann’s lab) (Yang et al., 2023a) and CRISPRi-depleted of individual ISR kinases (HRI, PKR, PERK, GCN2; a kind gift from Martin Kampmann’s lab at UCSF) (Guo et al., 2020), and MEFmtGFP (a kind gift from Peter Schultz) (Wang et al., 2012) were all cultured at 37°C and 5% CO2 in DMEM (Corning-Cellgro) supplemented with 10% fetal bovine serum (FBS, Gibco), 2 mM l-glutamine (Gibco), 100 U/mL penicillin, and 100 mg/mL streptomycin (Gibco).
Primary fibroblast cells were isolated from partial thickness skin biopsy, as previously described (Sharma et al., 2021b; Martens et al., 2020), from a patient who provided written informed consent for research studies using human tissues (University of Calgary Conjoint Research Ethics Board REB17-0850). Cells were cultured in Medium Essential Media (11095080, Gibco), supplemented with 10% FBS (12483020, Gibco). Cells were maintained at 37°C and 5% CO2. Clinical information regarding this participant was previously reported and included ataxia, optic atrophy, and sensorineural hearing loss (Sharma et al., 2021b). Exome sequencing in this participant identified a homozygous c.1241A>T variant in MFN2 (predicted to cause p.(Asp414Val)) and no other pathogenic variants.
Compounds and reagents
Request a detailed protocolThe compounds used in this study were purchased from the following sources: thapsigargin (Tg; Cat# 50-464-294 Fisher Scientific), ISRIB (Cat# SML0843, Sigma), CCCP (Cat# C2759, Sigma), BtdCPU (Cat# 32-489-210MG, Fisher), halofuginone (Cat#50-576-3001, Sigma), and oligomycin A (S1478, Selleck). The nucleoside mimetic library was purchased from Chem Div. Hit compounds were repurchased from Chem Div.
Measurements of ISR activation in ATF4-reporter cell lines
Request a detailed protocolHEK293 cells stably expressing the ATF4-FLuc, HSE-Fluc, or the ARE-Fluc reporter were seeded at a density of 15,000 cells per well in 384-well white plates with clear bottoms (Greiner). The following day, cells were treated with the indicated compound in triplicate at the indicated concentration for 8 hr. After treatment, an equal volume of Promega Bright-Glo substrate (Promega) was added to the wells and allowed to incubate at room temperature for 10 min. Luminescence was then measured using an Infinite F200 PRO plate reader (Tecan) with an integration time of 1000 ms. This assay was used to both screen the nucleoside mimetic library in triplicate and monitor the activity of hit compounds. HEK293 cells expressing the XBP1-RLuc reporter were tested using an analogous approach to that described above, monitoring RLuc activity using Renilla-Glo reagent (Promega), as previously described (Grandjean et al., 2020).
HEK293 cells stably expressing the ATF4-mApple reporter and CRISPRi-depleted of specific ISR kinases were seeded at a density of 300,000 cells per well in six-well TC-treated flat-bottom plates (Genesee Scientific). The cells were treated the next day for 16 hr with the compound at the indicated concentration. Cells were then washed twice with phosphate-buffered saline (PBS) and dissociated using TrypLE Express (Thermo Fisher). The cells were then resuspended in PBS and 5% FBS to neutralize the enzymatic reaction. Flow cytometry was performed on a Bio-Rad ZE5 Cell Analyzer monitoring mAPPLE fluorescence (568/592 nm) using the 561 nm green-yellow laser in combination with the 577/15 filter. Analysis was performed using FlowJo Software (BD Biosciences).
Quantitative PCR
Request a detailed protocolThe relative mRNA expression of target genes was measured using quantitative RT-PCR. Cells were treated as indicated and then washed with PBS (Gibco). RNA was extracted using Quick-RNA MiniPrepKit (Zymo Research) according to the manufacturer’s protocol. RNA (500 ng) was then converted to cDNA using the High-Capacity Reverse Transcription Kit (Applied Biosystems). qPCR reactions were prepared using Power SYBR Green PCR Master Mix (Applied Biosystems), and primers (below) were obtained from Integrated DNA Technologies. Amplification reactions were run in an ABI 7900HT Fast Real Time PCR machine with an initial melting period of 95°C for 5 min and then 45 cycles of 10 s at 95°C, 30 s at 60°C.
qPCR primers
Request a detailed protocolForward | Reverse | |
---|---|---|
M. musculus Chac1 | TGACCCTCCTTGAAGACCGTGA | AGTGTCATAGCCACCAAGCACG |
M. musculus Rplp2 | TGTCATCGCTCAGGGTGTTG | AAGCCAAATCCCATGTCGTC |
M. musculus Asns | CCAAGTTCAGTATCCTCTCC | TAATTTGCCACCTTTCTAGC |
H. sapiens RPLP0 | CCACGCTGCTGAACATGC | TCGAACACCTGCTGGATGAC |
H. sapiens ASNS | ATCACTGTCGGGATGTACCC | TGATAAAAGGCAGCCAATCC |
H. sapiens CHAC1 | GTGGTGACGCTCCTTGAAGA | TTCAGGGCCTTGCTTACCTG |
H. sapiens HSPA1A | GCTGATGATGGGGTTAACA | GGAGGCGGAGTACA |
H. sapiens NQO1 | GCCTCCTTCATGGCATAGTT | GGACTGCACCAGAGCCAT |
Fluorescence microscopy
Request a detailed protocolMEFmtGFP were seeded at a density of 15,000 cells/well in eight-chamber slides (Ibidi) coated with poly-d-lysine (Sigma) (Wang et al., 2012). The next day cells were treated with the indicated dose of compound for the indicated time. After treatment, cells were imaged on a Zeiss LSM 880 Confocal Laser Scanning Microscope equipped with a full incubation chamber for regulating temperature and CO2 during live-cell imaging.
Patient fibroblasts were seeded at 70,000 cells per dish in 35 mm dishes with 20 mm glass bottoms (D35-20-1.5-N, Cellvis) for live-cell imaging. After 24 hr, the compound treatments were administered at the dosage and for the time points indicated in the figure legends. The mitochondrial network in patient fibroblasts was visualized using 100 nM MitoTracker Green (M7514, Life Technologies) (Kumar et al., 2001) for 45 min, following washing three times with culture media, according to the manufacturer’s instructions. The Z-stack images were acquired of patient fibroblasts using an Olympus Spinning Disc Confocal System (Olympus SD OSR) equipped with the Olympus UPlanApo 60XTIRF/1.50 Oil Objective using the CellSense Dimensions software. Acquired Z-stacks were analyzed using AI Machine Learning Segmentation (Imaris), as detailed below.
Quantification of mitochondrial morphology
Request a detailed protocolThe Z-stack confocal images were processed in FIJI to reduce the background noise and enhance the fluorescent signal. The processed images are then introduced into the developed quantification pipeline in Imaris imaging software. In this approach, mitochondria are segmented in 3D using the ‘Surfaces’ module with a machine-learning algorithm that has been iteratively trained to detect foreground and background pixels in each Z-stack, filling blank holes within segments to generate 3D surfaces. The generated surfaces are filtered to include those above a minimum threshold of 250 voxels. While direct length measurements cannot be obtained through the Imaris surface module, indirect measurements of mitochondrial length are inferred from three separate calculations, including (1) object-oriented bounding box axis, (2) ellipsoid axis length, and (3) object sphericity (see Figure 2—figure supplement 1A). The object-oriented bounding box axis is calculated by measuring the length of the longest or principal bounding-box length of the smallest object-oriented rectangular box that encloses each 3D segmentation. The ellipsoid axis length is calculated by measuring the length of the longest or principal axis of each 3D segmentation. Sphericity is calculated by dividing the longest axis of each 3D segmentation by the length of the perpendicular axis.
Statistical methods
Request a detailed protocolData are presented as mean ± SEM or as violin plots showing the mean and quartiles for the indicated number of measurements. Outliers were removed from datasets describing bounding box length and principal axis length, as appropriate, using the ROUT outlier test in PRISM 10 (GraphPad, San Diego, CA). Normality of datasets from our imaging studies was tested in PRISM 10 (GraphPad) using D’Agostino & Pearson, Anderson–Darling, Shapiro–Wilk, and Kolmogorov–Smirnov tests. Statistics were calculated in PRISM 10 (GraphPad) and analyzed by one‐way ANOVA with Tukey’s multiple correction test, Kruskal–Wallis or Mann–Whitney tests for data exhibiting a non-normal distribution, as indicated in the accompanying figure legends. Indications of nonsignificant interactions were generally omitted for clarity.
Materials availability statement
Request a detailed protocolAll materials detailed in this article can be provided by the authors upon reasonable request or purchased from the indicated supplier.
Data availability
Source data files for Figure 1, Figure 2, Figure 3, Figure 4, Figure 1-figure supplement 1, Figure 2-figure supplement 1, Figure 3-figure supplement 1, and Figure 4 - figure supplement 1 contain the numerical data used to generate the corresponding figures.
References
-
Charcot-Marie-tooth disease type 2A: An update on pathogenesis and therapeutic perspectivesNeurobiology of Disease 193:106467.https://doi.org/10.1016/j.nbd.2024.106467
-
Folding the mitochondrial UPR into the integrated stress responseTrends in Cell Biology 30:428–439.https://doi.org/10.1016/j.tcb.2020.03.001
-
Inhibition of Drp1 ameliorates synaptic depressionAbeta Deposition, and Cognitive Impairment in an Alzheimer’s Disease Model. J Neurosci 37:5099–5110.https://doi.org/10.1523/JNEUROSCI.2385-16.2017
-
Quantifying organellar ultrastructure in cryo-electron tomography using a surface morphometrics pipelineThe Journal of Cell Biology 222:e202204093.https://doi.org/10.1083/jcb.202204093
-
Mechanistic and therapeutic role of Drp1 in the pathogenesis of Alzheimer’s diseaseThe European Journal of Neuroscience 56:5516–5531.https://doi.org/10.1111/ejn.15611
-
Targeting dynamin-related protein-1 as a potential therapeutic approach for mitochondrial dysfunction in Alzheimer’s diseaseBiochimica et Biophysica Acta - Molecular Basis of Disease 1869:166798.https://doi.org/10.1016/j.bbadis.2023.166798
-
Small-molecule proteostasis regulators for protein conformational diseasesNature Chemical Biology 8:185–196.https://doi.org/10.1038/nchembio.763
-
Activation of Gcn2 by small molecules designed to be inhibitorsThe Journal of Biological Chemistry 299:104595.https://doi.org/10.1016/j.jbc.2023.104595
-
Mitochondrial dynamics and its involvement in diseaseAnnual Review of Pathology 15:235–259.https://doi.org/10.1146/annurev-pathmechdis-012419-032711
-
Chemical genetics identify eIF2α kinase heme-regulated inhibitor as an anticancer targetNature Chemical Biology 7:610–616.https://doi.org/10.1038/nchembio.613
-
Mitochondrial dynamics in health and disease: mechanisms and potential targetsSignal Transduction and Targeted Therapy 8:333.https://doi.org/10.1038/s41392-023-01547-9
-
Mitochondrial proteases: multifaceted regulators of mitochondrial plasticityAnnual Review of Biochemistry 89:501–528.https://doi.org/10.1146/annurev-biochem-062917-012739
-
DELE1 tracks perturbed protein import and processing in human mitochondriaNature Communications 13:1853.https://doi.org/10.1038/s41467-022-29479-y
-
Mitochondrial calcium exchange in physiology and diseasePhysiological Reviews 102:893–992.https://doi.org/10.1152/physrev.00041.2020
-
The cell biology of mitochondrial membrane dynamicsNature Reviews. Molecular Cell Biology 21:204–224.https://doi.org/10.1038/s41580-020-0210-7
-
Pharmacologic IRE1/XBP1s activation confers targeted ER proteostasis reprogrammingNature Chemical Biology 16:1052–1061.https://doi.org/10.1038/s41589-020-0584-z
-
Inhibition of mitochondrial fragmentation diminishes Huntington’s disease-associated neurodegenerationThe Journal of Clinical Investigation 123:5371–5388.https://doi.org/10.1172/JCI70911
-
Transmission of cell stress from endoplasmic reticulum to mitochondria: enhanced expression of Lon proteaseThe Journal of Cell Biology 157:1151–1160.https://doi.org/10.1083/jcb.200108103
-
Receptor-mediated Drp1 oligomerization on endoplasmic reticulumThe Journal of Cell Biology 216:4123–4139.https://doi.org/10.1083/jcb.201610057
-
Inhibition of Drp1/Fis1 interaction slows progression of amyotrophic lateral sclerosisEMBO Molecular Medicine 10:e8166.https://doi.org/10.15252/emmm.201708166
-
Halofuginone and other febrifugine derivatives inhibit prolyl-tRNA synthetaseNature Chemical Biology 8:311–317.https://doi.org/10.1038/nchembio.790
-
Targeting of the c-Abl tyrosine kinase to mitochondria in the necrotic cell death response to oxidative stressThe Journal of Biological Chemistry 276:17281–17285.https://doi.org/10.1074/jbc.M101414200
-
Mitochondrial fission and fusion: a dynamic role in aging and potential target for age-related diseaseMechanisms of Ageing and Development 186:111212.https://doi.org/10.1016/j.mad.2020.111212
-
Mapping stress-responsive signaling pathways induced by mitochondrial proteostasis perturbationsMolecular Biology of the Cell 35:ar74.https://doi.org/10.1091/mbc.E24-01-0041
-
Rescue axonal defects by targeting mitochondrial dynamics in hereditary spastic paraplegiasNeural Regeneration Research 14:574–577.https://doi.org/10.4103/1673-5374.248108
-
Neurodegeneration risk factor, EIF2AK3 (PERK), influences tau protein aggregationThe Journal of Biological Chemistry 299:102821.https://doi.org/10.1016/j.jbc.2022.102821
-
Updated review of therapeutic strategies for Charcot-Marie-Tooth disease and related neuropathiesExpert Review of Neurotherapeutics 21:701–713.https://doi.org/10.1080/14737175.2021.1935242
-
Determinants and outcomes of mitochondrial dynamicsMolecular Cell 83:857–876.https://doi.org/10.1016/j.molcel.2023.02.012
-
Mitonuclear communication in homeostasis and stressNature Reviews. Molecular Cell Biology 17:213–226.https://doi.org/10.1038/nrm.2016.23
-
A mitochondrial iron-responsive pathway regulated by DELE1Molecular Cell 83:2059–2076.https://doi.org/10.1016/j.molcel.2023.05.031
-
Quality control of the mitochondrial proteomeNature Reviews Molecular Cell Biology 22:54–70.https://doi.org/10.1038/s41580-020-00300-2
-
The good and the bad of mitochondrial breakupsTrends in Cell Biology 29:888–900.https://doi.org/10.1016/j.tcb.2019.08.003
-
Activation of the integrated stress response by inhibitors of its kinasesNature Communications 14:5535.https://doi.org/10.1038/s41467-023-40823-8
-
Discovery of HC-7366: an orally bioavailable and efficacious GCN2 kinase activatorJournal of Medicinal Chemistry 67:5259–5271.https://doi.org/10.1021/acs.jmedchem.3c02384
-
Mitochondrial dynamics and metabolic regulationTrends in Endocrinology and Metabolism 27:105–117.https://doi.org/10.1016/j.tem.2015.12.001
-
A small molecule promotes mitochondrial fusion in mammalian cellsAngewandte Chemie 51:9302–9305.https://doi.org/10.1002/anie.201204589
-
DELE1 oligomerization promotes integrated stress response activationNature Structural & Molecular Biology 30:1295–1302.https://doi.org/10.1038/s41594-023-01061-0
-
Genetic profile and onset features of 1005 patients with Charcot-Marie-Tooth disease in JapanJournal of Neurology, Neurosurgery, and Psychiatry 90:195–202.https://doi.org/10.1136/jnnp-2018-318839
-
Tauopathy-associated PERK alleles are functional hypomorphs that increase neuronal vulnerability to ER stressHuman Molecular Genetics 27:3951–3963.https://doi.org/10.1093/hmg/ddy297
-
The role of impaired mitochondrial dynamics in MFN2-mediated pathologyFrontiers in Cell and Developmental Biology 10:858286.https://doi.org/10.3389/fcell.2022.858286
Article and author information
Author details
Funding
National Institutes of Health (NS095892)
- R Luke Wiseman
National Institutes of Health (NS125674)
- Danielle A Grotjahn
- R Luke Wiseman
National Institutes of Health (AG081061)
- Kelsey R Baron
National Science Foundation Graduate Research Fellowship Program
- Samantha Oviedo
- Rama Aldakhlallah
Hotchkiss Brain Institute, University of Calgary
- Mashiat Zaman
Canadian Institutes of Health Research
- Timothy E Shutt
The funders had no role in study design, data collection and interpretation, or the decision to submit the work for publication.
Acknowledgements
We thank Jie Sun and Sergei Kutseikin for experimental support and Evan Powers for critical reading of the manuscript. We thank Kathy Spencer and Scott Henderson in the TSRI Microscopy Facility for their support on the confocal imaging and analysis described in this project. We would also like to thank Martin Kampmann (UCSF), Xiaoyan Guo (UConn), and Jonathan Lin (Stanford) for experimental resources and advice related to this project. This work was supported by the National Institutes of Health (NIH; NS095892, NS125674 to RLW), the Canadian Institutes of Health Research (TES), an NIH F30 Predoctoral Fellowship (AG081061 to KB), National Science Foundation Predoctoral Fellowships (to SO and RA), and the Hotchkiss Brain Institute International Recruitment Scholarship (MZ).
Version history
- Preprint posted:
- Sent for peer review:
- Reviewed Preprint version 1:
- Reviewed Preprint version 2:
- Version of Record published:
Cite all versions
You can cite all versions using the DOI https://doi.org/10.7554/eLife.100541. This DOI represents all versions, and will always resolve to the latest one.
Copyright
© 2024, Baron, Oviedo et al.
This article is distributed under the terms of the Creative Commons Attribution License, which permits unrestricted use and redistribution provided that the original author and source are credited.
Metrics
-
- 1,151
- views
-
- 82
- downloads
-
- 3
- citations
Views, downloads and citations are aggregated across all versions of this paper published by eLife.
Download links
Downloads (link to download the article as PDF)
Open citations (links to open the citations from this article in various online reference manager services)
Cite this article (links to download the citations from this article in formats compatible with various reference manager tools)
Further reading
-
- Cancer Biology
- Cell Biology
Cell crowding causes high-grade breast cancer cells to become more invasive by activating a molecular switch that causes the cells to shrink and spread.
-
- Cell Biology
Overnutrition engenders the expansion of adipose tissue and the accumulation of immune cells, in particular, macrophages, in the adipose tissue, leading to chronic low-grade inflammation and insulin resistance. In obesity, several proinflammatory subpopulations of adipose tissue macrophages (ATMs) identified hitherto include the conventional ‘M1-like’ CD11C-expressing ATM and the newly discovered metabolically activated CD9-expressing ATM; however, the relationship among ATM subpopulations is unclear. The ER stress sensor inositol-requiring enzyme 1α (IRE1α) is activated in the adipocytes and immune cells under obesity. It is unknown whether targeting IRE1α is capable of reversing insulin resistance and obesity and modulating the metabolically activated ATMs. We report that pharmacological inhibition of IRE1α RNase significantly ameliorates insulin resistance and glucose intolerance in male mice with diet-induced obesity. IRE1α inhibition also increases thermogenesis and energy expenditure, and hence protects against high fat diet-induced obesity. Our study shows that the ‘M1-like’ CD11c+ ATMs are largely overlapping with but yet non-identical to CD9+ ATMs in obese white adipose tissue. Notably, IRE1α inhibition diminishes the accumulation of obesity-induced metabolically activated ATMs and ‘M1-like’ ATMs, resulting in the curtailment of adipose inflammation and ensuing reactivation of thermogenesis, without augmentation of the alternatively activated M2 macrophage population. Our findings suggest the potential of targeting IRE1α for the therapeutic treatment of insulin resistance and obesity.