Vascular Development: Enhancing our understanding of endothelial cells
The blood vessels in our bodies are either arteries, veins or capillaries. Arteries transport blood away from the heart, veins carry it back, and capillaries connect arteries and veins, while also facilitating the exchange of gases and nutrients between the blood and different organs (Augustin and Koh, 2017). The inner layer of every blood vessel is formed by endothelial cells, but what factors determine whether a newly formed endothelial cell becomes part of an artery or a vein or a capillary?
The formation of blood vessels during embryonic development begins with the differentiation of mesodermal cells into endothelial cells, which then generate new blood vessels through a process known as vasculogenesis (Choi et al., 1998). Subsequently, additional blood vessels arise from pre-existing ones via angiogenesis (Adams and Alitalo, 2007). Although the first arterial endothelial cells are produced during vasculogenesis (Chong et al., 2011), studies performed on humans, mice and zebrafish have shown that most arterial endothelial cells originate from venous endothelial cells (Hou et al., 2022).
The differences between arterial, venous and capillary endothelial cells have been the subject of much research, but the substantial changes in gene expression that are required for venous endothelial cells to become arterial endothelial cells have received less attention. Now, in eLife, Sarah De Val of the University of Oxford and colleagues – including Svanhild Nornes as first author – report that they have identified a list of genetic enhancers and transcription factors that have a crucial role in this process (Nornes et al., 2025). Enhancers are non-coding regions of DNA that are involved in regulating gene expression (Thomas and Buecker, 2023), while transcription factors are proteins that regulate gene expression by binding to enhancers (Panigrahi and O’Malley, 2021).
Nornes et al. studied the genomic localization of eight genes known to be highly enriched in arterial endothelial cells by analyzing five published datasets that contained detailed information on enhancer-associated marks in the DNA (Figure 1). This analysis identified 41 putative arterial enhancers, three of which had been previously studied in vivo. Subsequent experiments on genetically-engineered zebrafish that used green fluorescent protein (GFP) to report on gene expression, showed that sixteen of the enhancers drove gene expression in blood vessels, with fifteen specifically driving gene expression in arteries. Moreover, the researchers identified at least one enhancer for each of the eight genes. Further experiments on embryonic mice showed that these enhancers also drove gene expression in arteries.
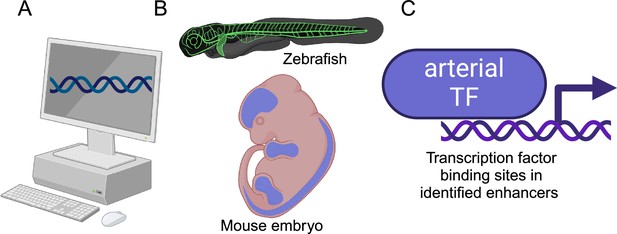
Investigating arterial gene expression.
(A) Nornes et al. used computational methods to identify 41 potential enhancers for eight genes that are highly enriched in arterial endothelial cells (Acvrl1, Cxcr4, Cxcl12, Efnb2, Gja4, Gja5, Nrp1, and Unc5b). (B) In vivo experiments on zebrafish and mice confirmed that 15 of these enhancers were involved in the regulation of arterial gene expression. (C) Subsequent experiments identified potential transcription factors (TFs) that could bind to these enhancers (see text). Created in BioRender.com.
This combination of zebrafish and mouse experiments confirmed that Nornes et al. had successfully identified a cohort of enhancers capable of directing gene expression to arterial endothelial cells. Additionally, they explored the potential transcription factors that might bind to these enhancers. The researchers identified a number of transcription factors – such as ETS, SOXF and FOX – that are enriched in arterial endothelial cells, although they can also be found in venous endothelial cells. The researchers also found that the transcription factor MEF2 binds to enhancers in a subset of arterial endothelial cells associated with angiogenesis. Moreover, evidence for the binding of another transcription factor – RBPJ – suggests that the Notch signaling pathway plays a role in regulating the expression of arterial-related genes.
The work of Nornes et al. – notably, the insights it provides into the regulatory mechanisms that define arterial identity, and the identification of the various arterial enhancers and the transcription factor binding sites for these enhancers – will be useful to researchers studying vascular patterning in general, as well as how its disruption can lead to abnormal vascular development and arteriovenous malformations. These malformations can result in blood flowing directly from arteries to veins without passing through any capillaries (Atri et al., 2013). If this happens in the brain, it can cause ischemia, intracerebral hemorrhage, disability, stroke and death (Friedlander, 2007), so there is a pressing need for a better understanding of processes underpinning the differentiation of blood vessels.
References
-
Molecular regulation of angiogenesis and lymphangiogenesisNature Reviews Molecular Cell Biology 8:464–478.https://doi.org/10.1038/nrm2183
-
Endothelial signaling and the molecular basis of arteriovenous malformationCellular and Molecular Life Sciences 71:867–883.https://doi.org/10.1007/s00018-013-1475-1
-
Stepwise arteriovenous fate acquisition during mammalian vasculogenesisDevelopmental Dynamics 240:2153–2165.https://doi.org/10.1002/dvdy.22706
-
Clinical practice arteriovenous malformations of the brainNew England Journal of Medicine 356:2704–2712.https://doi.org/10.1056/NEJMcp067192
Article and author information
Author details
Publication history
Copyright
© 2025, Luxán
This article is distributed under the terms of the Creative Commons Attribution License, which permits unrestricted use and redistribution provided that the original author and source are credited.
Metrics
-
- 366
- views
-
- 42
- downloads
-
- 0
- citations
Views, downloads and citations are aggregated across all versions of this paper published by eLife.
Download links
Downloads (link to download the article as PDF)
Open citations (links to open the citations from this article in various online reference manager services)
Cite this article (links to download the citations from this article in formats compatible with various reference manager tools)
Further reading
-
- Cancer Biology
- Developmental Biology
Missense ‘hotspot’ mutations localized in six p53 codons account for 20% of TP53 mutations in human cancers. Hotspot p53 mutants have lost the tumor suppressive functions of the wildtype protein, but whether and how they may gain additional functions promoting tumorigenesis remain controversial. Here, we generated Trp53Y217C, a mouse model of the human hotspot mutant TP53Y220C. DNA damage responses were lost in Trp53Y217C/Y217C (Trp53YC/YC) cells, and Trp53YC/YC fibroblasts exhibited increased chromosome instability compared to Trp53-/- cells. Furthermore, Trp53YC/YC male mice died earlier than Trp53-/- males, with more aggressive thymic lymphomas. This correlated with an increased expression of inflammation-related genes in Trp53YC/YC thymic cells compared to Trp53-/- cells. Surprisingly, we recovered only one Trp53YC/YC female for 22 Trp53YC/YC males at weaning, a skewed distribution explained by a high frequency of Trp53YC/YC female embryos with exencephaly and the death of most Trp53YC/YC female neonates. Strikingly, however, when we treated pregnant females with the anti-inflammatory drug supformin (LCC-12), we observed a fivefold increase in the proportion of viable Trp53YC/YC weaned females in their progeny. Together, these data suggest that the p53Y217C mutation not only abrogates wildtype p53 functions but also promotes inflammation, with oncogenic effects in males and teratogenic effects in females.
-
- Developmental Biology
Stem cell self-renewal often relies on asymmetric fate determination governed by niche signals and/or cell-intrinsic factors but how these regulatory mechanisms cooperate to promote asymmetric fate decision remains poorly understood. In adult Drosophila midgut, asymmetric Notch (N) signaling inhibits intestinal stem cell (ISC) self-renewal by promoting ISC differentiation into enteroblast (EB). We have previously shown that epithelium-derived Bone Morphogenetic Protein (BMP) promotes ISC self-renewal by antagonizing N pathway activity (Tian and Jiang, 2014). Here, we show that loss of BMP signaling results in ectopic N pathway activity even when the N ligand Delta (Dl) is depleted, and that the N inhibitor Numb acts in parallel with BMP signaling to ensure a robust ISC self-renewal program. Although Numb is asymmetrically segregated in about 80% of dividing ISCs, its activity is largely dispensable for ISC fate determination under normal homeostasis. However, Numb becomes crucial for ISC self-renewal when BMP signaling is compromised. Whereas neither Mad RNA interference nor its hypomorphic mutation led to ISC loss, inactivation of Numb in these backgrounds resulted in stem cell loss due to precocious ISC-to-EB differentiation. Furthermore, we find that numb mutations resulted in stem cell loss during midgut regeneration in response to epithelial damage that causes fluctuation in BMP pathway activity, suggesting that the asymmetrical segregation of Numb into the future ISC may provide a fail-save mechanism for ISC self-renewal by offsetting BMP pathway fluctuation, which is important for ISC maintenance in regenerative guts.